Fluorescent Spectrophotometry: Principles and Advances
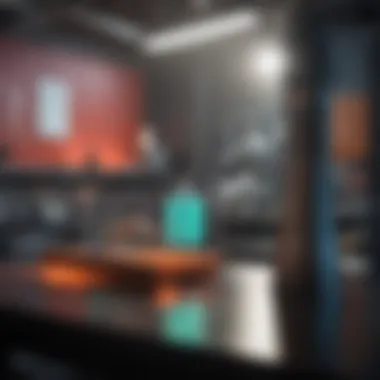
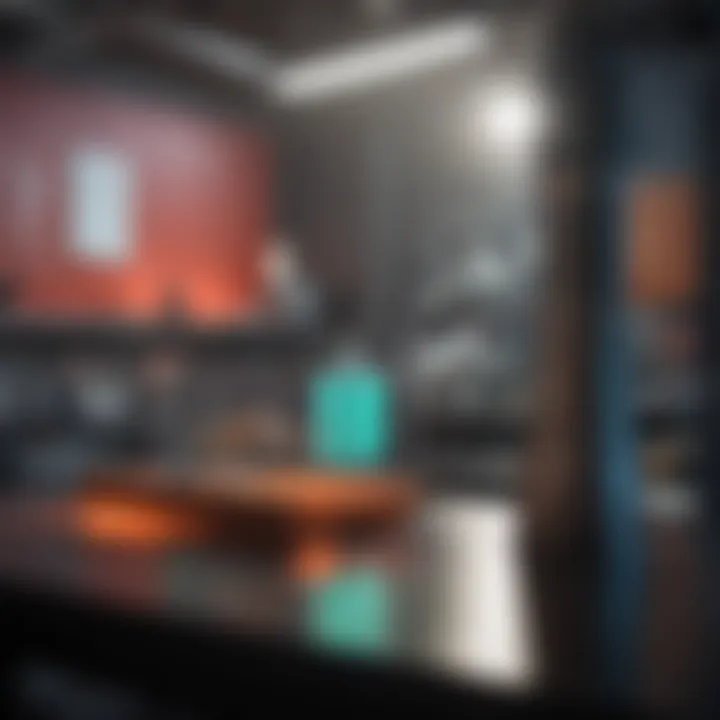
Intro
Fluorescent spectrophotometry is an essential analytical technique integral to various scientific fields, including chemistry, biology, and environmental science. Its ability to detect and quantify fluorescent substances plays a critical role in studying molecular interactions and monitoring environmental changes. This method relies on the emission of light from a sample after it has absorbed photons, making it a powerful tool for research and practical applications.
Understanding the principles and mechanisms behind fluorescent spectrophotometry is essential for both newcomers and seasoned researchers. The depth of insights gained from this technique can be invaluable when applied correctly. The equipment used in this method, along with the data analysis techniques employed, come together to form a framework that can address complex scientific inquiries. Moreover, advancements in technology continuously shape the practice, enabling more precise and accurate measurements. This article elaborates on these aspects, providing a comprehensive overview while also discussing the challenges that arise in real-world scenarios.
As fluorescent spectrophotometry becomes more popular across numerous domains, it also becomes increasingly important to explore its underlying principles, practical applications, and the innovations that define its future.
Intro to Fluorescent Spectrophotometry
Fluorescent spectrophotometry is a key analytical technique in various scientific disciplines. Its importance lies in the ability to detect and measure light emitted from a substance after it absorbs light or other electromagnetic radiation. This technique is vital in fields such as biology, chemistry, and environmental science, where understanding molecular behavior is crucial. The growing application of fluorescent spectrophotometry helps in unraveling complex interactions at a molecular level, offering insights into diverse research areas.
In this article, we explore several important elements associated with fluorescent spectrophotometry. The discussion covers its fundamental principles, instrumentation, and a wide range of applications. Each aspect is interconnected, contributing to the broader understanding of how this technique can be utilized effectively. Additional focus will be on advancements made in recent years and the challenges that researchers face. By grasping the significance of these elements, one can appreciate the depth and utility of fluorescent spectrophotometry in modern science.
Definition and Overview
Fluorescent spectrophotometry involves measuring the intensity of fluorescence emitted from a sample when it is excited by a specific wavelength of light. This technique is distinguished from other forms of spectroscopy by its ability to detect very low concentrations of fluorescent molecules. The basic principle relies on the excitation of electrons within a molecule, leading to the emission of light as the electrons return to their ground state. The emitted light is then analyzed to determine various properties of the sample, such as concentration and purity.
In essence, this method is powerful for quantifying substances and studying physical and chemical properties of diverse materials. Its sensitivity and specificity make it suitable for analyzing biological samples, environmental pollutants, and material compositions.
Historical Background
The roots of fluorescent spectrophotometry can be traced back to the early 20th century. Innovations in the field began with the discovery of fluorescence, where scientists such as Stokes identified the shift in wavelength between absorbed and emitted light.
As technology advanced, so did the sophistication of fluorescent spectrometers. The introduction of electronic detectors, such as photomultiplier tubes, allowed for improved sensitivity and quicker data acquisition. Over the decades, continuous refinements have led to the development of portable and automated systems, broadening the accessibility of this technique to various research laboratories.
Today, fluorescent spectrophotometry enjoys a strong presence in the scientific community. The widespread use of this technique is a result of its efficiency and reliable performance, making it a cornerstone in both academic research and industrial applications.
Fundamental Principles
Understanding the fundamental principles of fluorescent spectrophotometry is crucial for the effective application of this analytical technique. These principles lay the groundwork for the processes, mechanisms, and variables involved in fluorescence. They offer insights into how molecules emit light after absorbing energy, which is central to various applications across scientific disciplines. The comprehension of these principles aids researchers in optimizing experiments and interpreting results accurately.
The Process of Fluorescence
Fluorescence is a photophysical process where a substance absorbs photons and then re-emits them. This begins when a molecule is excited by light, typically ultraviolet or blue light. Upon excitation, the electrons of the molecule are elevated to a higher energy level. After a brief period, known as the excited state, electrons return to their ground state, releasing energy in the form of light. The duration between absorption and re-emission is very short, generally in the range of nanoseconds.
The emitted light usually has a longer wavelength than the absorbed light. The conversion of energy during this process results in a specific color of light depending on the moleculeβs structure. Different molecules will exhibit unique fluorescence properties, which can be utilized for various applications, such as imaging or concentration measurements.
Stokes Shift
Stokes shift is a pivotal concept in fluorescent spectrophotometry. It refers to the difference between the wavelengths of the absorbed light and the emitted light. This phenomenon occurs due to the loss of energy through non-radiative processes after excitation. The Stokes shift can provide crucial information on the environment of the fluorescent molecule, indicating interactions with surrounding molecules or changes in fluid properties.
A greater Stokes shift is often advantageous in fluorescent applications since it minimizes overlap between the excitation and emission spectra. This reduces background interference, leading to better signal clarity and sensitivity in measurements. Understanding Stokes shift aids researchers in the selection of fluorescent markers for their specific applications.
Quantum Yield
Quantum yield defines the efficiency of the fluorescence process. It is the ratio of the number of photons emitted to the number of photons absorbed by the fluorescent molecules. In simpler terms, it measures how well a fluorescent compound converts absorbed light into emitted light. This variable is influenced by various factors, such as the molecular structure, environmental conditions, and the presence of quenching agents.
A high quantum yield is desirable for effective fluorescent applications as it ensures a stronger signal. Conversely, a low quantum yield can result in weak signals and may complicate data analysis. Understanding quantum yield is essential for optimizing experiments and is a critical factor in the design of new fluorescent probes and markers.
Key Takeaway: The fundamental principles of fluorescent spectrophotometry, including fluorescence mechanics, Stokes shift, and quantum yield, form the basis for its applications and effectiveness in scientific research.
Instrumentation
Instrumentation is crucial in fluorescent spectrophotometry because it defines the accuracy and reliability of the measurements obtained. The technology used in instruments can significantly affect the sensitivity, resolution, and overall performance. Researchers rely on precise instrumentation to obtain valid data for their experiments and applications. Therefore, understanding the components and types of fluorescent spectrophotometers, as well as the techniques for detection, is essential for advancing this field.
Components of Fluorescent Spectrophotometers
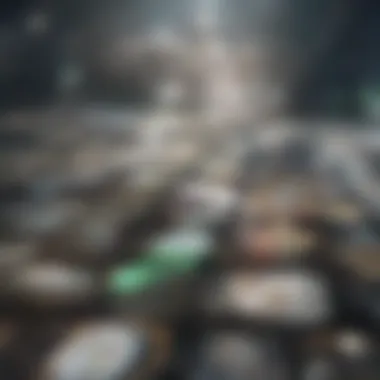
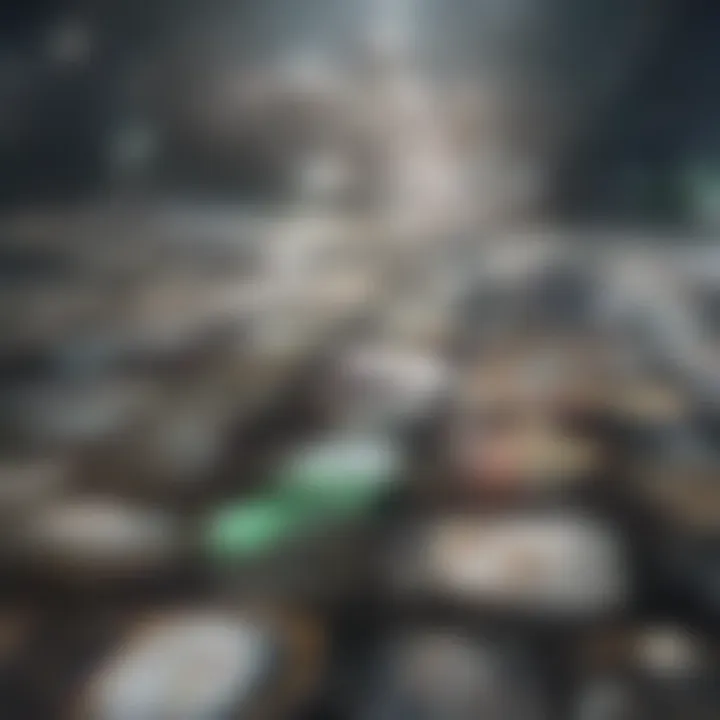
Fluorescent spectrophotometers consist of several key components, each playing a vital role in the operation.
- Light Source: Typically a high-intensity lamp, like a xenon lamp or LED, that provides the necessary excitation light. The choice of light source impacts the excitation wavelength and the overall sensitivity of the system.
- Monochromators: These devices filter the light into specific wavelengths to ensure that only the desired excitation light reaches the sample. Depending on the instrument design, this can enhance selectivity and reduce background noise.
- Sample Holder: Designed to securely contain the sample while allowing efficient light interaction. This component must be carefully designed to minimize any interference during measurements.
- Photodetector: This element detects the emitted fluorescence signal after the sample has been excited. Common detectors include photomultiplier tubes (PMTs) and avalanche photodiodes (APDs).
- Software Interface: Modern instruments commonly feature software for data acquisition and analysis, allowing researchers to interpret the results more effectively.
Types of Spectrophotometers
The types of fluorescent spectrophotometers vary based on design and application, leading to important distinctions.
Single Beam vs. Double Beam
Single beam spectrophotometers measure the fluorescence by passing the light through the sample and directly to the detector. The simplicity of this design makes it relatively easy to operate and maintain. However, it may suffer from drifting baseline measurements due to changes in the light source.
Double beam spectrophotometers, in contrast, split the excitation light between the sample and a reference beam. This setup provides a more stable baseline and compensates for fluctuations in the light source. The accuracy gained from this design makes it a popular choice in rigorous research environments. Despite its advantages, the added complexity often results in higher costs and maintenance.
Portable vs. Laboratory Spectrophotometers
Portable spectrophotometers are designed for field use, providing flexibility for on-site measurements. These instruments are typically compact and battery operated, making them a practical choice for environmental monitoring and other outdoor applications. The trade-off is often in their sensitivity and resolution compared to laboratory models.
Lab-based spectrophotometers, in contrast, offer enhanced performance and accuracy, suitable for controlled conditions. Their robust features allow for higher throughput and advanced data analysis. However, they are usually not as convenient for fieldwork.
Detection Techniques
Detection techniques play a vital role in fluorescent spectrophotometry. These methods help in distinguishing and quantifying the emitted light from various sources. The two primary techniques include:
- Fluorescence Lifetime Measurement: Involves measuring how long the fluorescent light lasts after an excitation pulse. This technique can provide information about the environment around the fluorescent molecules.
- Time-resolved Fluorescence: A method that enables differentiation between the fluorescence from the target molecules and any background interference. It is particularly useful in complex samples, enhancing the accuracy of the results.
"The choice of detection technique directly influences the interpretation of spectral data and the conclusions drawn in research."
In summary, instrumentation forms the backbone of fluorescent spectrophotometry, impacting every aspect of data acquisition and analysis. Understanding the components, various types, and detection methods leads to better insights and improved research outcomes.
Applications in Scientific Research
Fluorescent spectrophotometry plays a pivotal role in various branches of scientific research. Its capacity to provide detailed information about molecular structures and interactions makes it a vital tool for researchers. The technique offers unique advantages such as high sensitivity and specificity, which are critical for advancing our understanding in many fields, including biology, environmental science, and materials science. Consequently, researchers can obtain quantitative data on concentrations of substances in complex matrices, facilitating the detection and analysis of biomolecules, pollutants, and nanomaterials.
Biological and Biomedical Applications
Cellular Imaging
Cellular imaging is a significant application in the field of fluorescent spectrophotometry. It allows for the visualization of live cells, providing insights into cellular structures, dynamics, and interactions. A key characteristic of cellular imaging is its ability to observe real-time processes within living organisms. This is particularly beneficial for understanding diseases and cellular responses to treatments. The unique feature of this approach is the use of fluorescent markers that bind specifically to biological targets, enabling researchers to track molecular processes in situ. However, there are disadvantages, including potential phototoxic effects on cells and concerns about the stability of the fluorescent labels over extended observation periods.
Protein Interaction Studies
Protein interaction studies are another crucial application of fluorescent spectrophotometry, essential for exploring complex biological mechanisms. This technique allows researchers to monitor interactions between proteins, thereby contributing to the understanding of signaling pathways and disease mechanisms. The key characteristic of this approach lies in its ability to provide quantitative data on binding affinities and kinetics. This makes it a popular choice in drug discovery and development. The unique aspect of protein interaction studies, particularly using methods like FRET (FΓΆrster Resonance Energy Transfer), is its capacity to measure interactions at the nanometer scale. Nevertheless, challenges remain, such as the requirement for precise experimental conditions and the potential for background signals that can complicate data interpretation.
Environmental Monitoring
Detection of Pollutants
Detection of pollutants using fluorescent spectrophotometry represents a vital application in environmental monitoring. This method allows for the sensitive detection of a wide variety of contaminants, including heavy metals and organic compounds, aiding in environmental protection efforts. A key advantage of this technique is its ability to determine low concentrations of pollutants in water and soil samples quickly. The unique feature here is the use of specific fluorescent probes that can selectively bind to target pollutants, ensuring accurate detection. However, there are limitations, such as interference from other substances in complex environmental samples, which can affect the accuracy of measurements.
Water Quality Assessment
Water quality assessment is critical in managing and safeguarding water resources. Fluorescent spectrophotometry provides a powerful tool for analyzing the presence of microorganisms and toxins in water bodies. Its key characteristic is rapid analysis, allowing for the quick identification of harmful substances. This feature is particularly beneficial in both freshwater and marine environments. By employing fluorescent techniques, researchers can gather quantitative data that is crucial for environmental regulations. The disadvantage, however, includes the necessity of appropriate sample preparation and potential matrix effects that might hinder accurate readings.
Material Science
Characterization of Nanomaterials
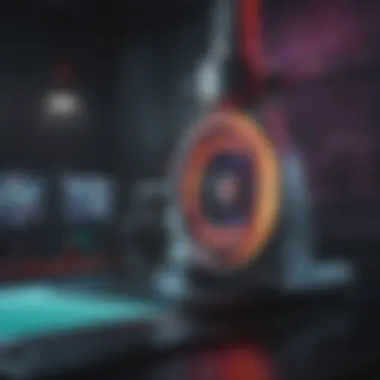
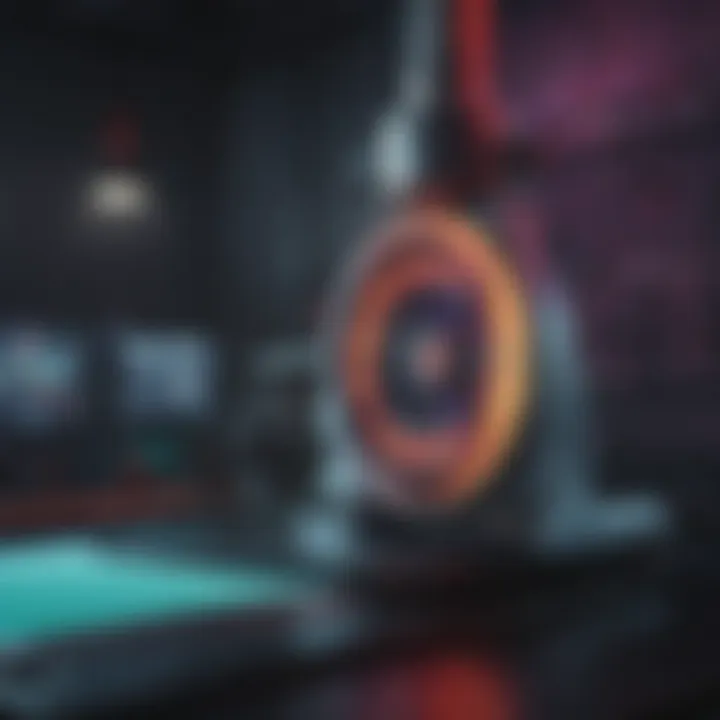
Characterization of nanomaterials is another dynamic application of fluorescent spectrophotometry. This area focuses on examining the unique optical properties of nanomaterials, such as quantum dots and nanorods. A key characteristic of this technique is its ability to provide information about the size, shape, and distribution of nanoparticles. This is beneficial for researchers aiming to understand the fundamental properties that influence the performance of nanomaterials in various applications. The unique feature is its application in assessing the photostability and luminescence of these materials. However, the complexity of nanomaterials can pose challenges, including difficulties in reproducibility and the need for advanced instrumentation.
Polymer Studies
Polymer studies benefit greatly from fluorescent spectrophotometry by enabling researchers to understand polymer behavior and properties at the molecular level. The key characteristic here is the sensitivity to changes in the environment around the polymer chains, which can indicate phase changes or interactions with additives. This makes it a popular choice for studying the effects of different conditions on polymer performance in applications like coatings and biomedical devices. The unique aspect of polymer studies using fluorescent techniques is the possibility to monitor the dynamics of polymerization processes. However, challenges must be considered, such as the interpretation of results that could be confounded by the presence of impurities or variations in sample preparation.
The diverse applications of fluorescent spectrophotometry across scientific research highlight its versatility and importance in advancing knowledge and addressing real-world problems.
Data Analysis Techniques
Data analysis techniques are crucial in the effective utilization of fluorescent spectrophotometry. This field generates a large amount of data from various experiments, requiring systematic approaches for interpretation and analysis. Understanding spectral data is essential for extracting meaningful information from fluorescence measurements. These techniques not only enhance the accuracy of results but also allow researchers to draw significant conclusions from their findings.
Spectral Data Interpretation
The interpretation of spectral data involves analyzing the emission spectra obtained from samples. Each spectrum is unique, reflecting the specific properties of the molecules present. Researchers need to understand how to differentiate between various peaks and their corresponding wavelengths to draw accurate conclusions about molecular characteristics. Key considerations in this process include:
- Baseline correction: This corrects any distortions in signal originating from instrumental noise or background fluorescence.
- Peak assignment: Accurately identifying peaks allows determination of specific molecular species in the sample.
Effective spectral data interpretation can reveal insights about molecular interactions, concentrations, and dynamics. It is the first step toward a deeper understanding of how substances behave under fluorescent light.
Computational Methods
Computational methods have revolutionized the analysis of fluorescent spectrophotometry data. They support complex data handling and enhance the analytical capabilities of researchers. These methods encompass various algorithms and models that can process large datasets efficiently.
Machine Learning Applications
Machine learning is a powerful tool in data analysis for fluorescent spectrophotometry. Its ability to learn patterns from data and make predictions is a significant advantage. Key characteristics include:
- Data-driven insights: Machine learning utilizes existing data to develop predictive models that can optimize experimental processes.
- Automation: It can automate data analysis, significantly reducing time and effort required in interpretation.
One unique feature of machine learning is its adaptability. Models can be trained on specific datasets to improve accuracy over time. However, there are also disadvantages. The need for large datasets to train models can be a limitation for some applications. Overall, machine learning is a beneficial and growing field in data analysis, driving advancements in fluorescent spectrophotometry.
Spectral Deconvolution Techniques
Spectral deconvolution is another essential computational method. It aims to separate overlapping spectral features, providing a clearer understanding of complex mixtures. This technique helps researchers isolate specific emissions from molecules that may not be distinguishable otherwise. Important aspects include:
- Resolution enhancement: By resolving overlapping spectra, it improves the overall clarity of the data.
- Quantitative analysis: Enables precise quantification of components within a sample, which is vital in many scientific fields.
A unique feature of this technique is its ability to process data obtained from sophisticated experiments. However, its implementation can involve complex algorithms requiring careful parameter selection. While spectral deconvolution offers significant advantages for obtaining detailed molecular information, it demands a solid understanding of the underlying principles to be used effectively.
Recent Advancements
The field of fluorescent spectrophotometry has witnessed various advancements in recent years, enhancing its capabilities and broadening its applications. These improvements not only heighten the efficiency of the techniques involved but also expand the scientific inquiry options available. The significance of these advancements lies in their potential to generate more accurate data, improve experimental outcomes, and ultimately contribute to discoveries across multiple disciplines.
Improvements in Sensitivity and Resolution
Recent developments have focused on enhancing the sensitivity and resolution of fluorescent spectrophotometers. Sensitivity refers to the instrument's ability to detect low concentrations of fluorescent molecules. Improvements in detector technology, such as the incorporation of ultra-sensitive photomultiplier tubes, allow for the detection of single-molecule fluorescence. This capability is critical in studies involving rare events in complex biological systems, where detecting subtle interactions can provide profound insights.
Moreover, advancements in optical components have led to improvements in resolution. Improved optics help in minimizing background noise, allowing for clearer and more distinct readings. Enhanced filters and monochromators enable better separation of fluorescence signals from overlapping spectra, leading to more accurate interpretations of results. This is particularly useful in environments where multiple fluorescent markers are used simultaneously, such as in cellular imaging studies.
Emerging Fluorescent Probes
The surge in the development of novel fluorescent probes marks another critical advancement in this field. Emerging fluorescent probes are designed to provide specific responses to distinct molecular environments, offering greater insights into biological processes. These include genetically encoded fluorescent proteins, small molecule probes, and nanoparticle-based systems, each designed to target specific cellular components or pathways.
Such probes enhance the functionality of fluorescent spectrophotometry by enabling the study of dynamic processes in real-time. They can indicate changes in cellular environments, such as pH levels or the presence of specific ions. Additionally, the development of multicolor probes allows researchers to track multiple processes within a single sample, leading to a more comprehensive view of cellular behavior.
"The integration of emerging fluorescent probes with advanced detection methods enhances the potential for groundbreaking discoveries across various research domains."
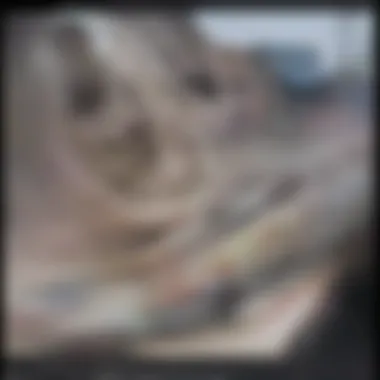
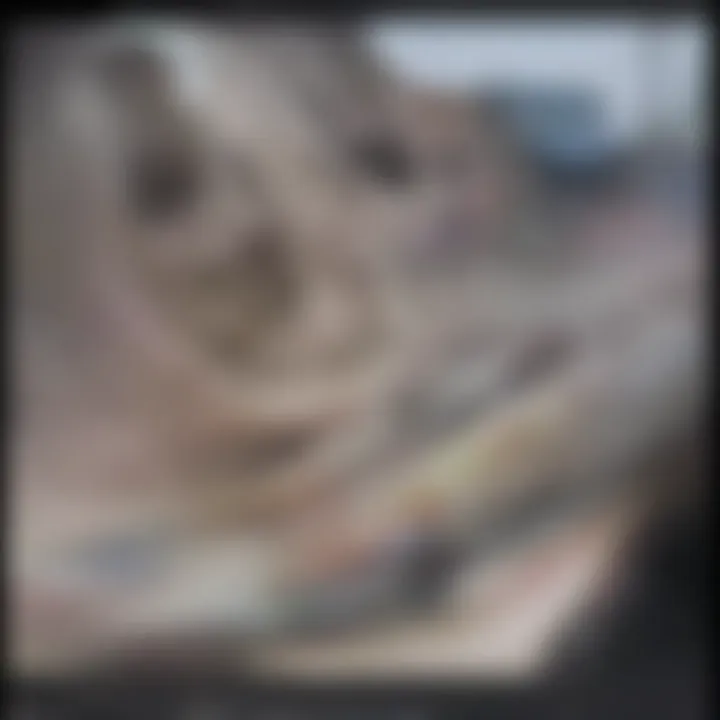
Challenges in Fluorescent Spectrophotometry
Fluorescent spectrophotometry, while a powerful tool in various scientific domains, is not without its challenges. Understanding these challenges is crucial for researchers and practitioners in the field. These challenges can significantly impact the reliability and accuracy of results, thus highlighting the importance of addressing them effectively.
Photobleaching and background interference are two of the most prominent issues faced in fluorescent spectrophotometry. Both factors can mislead interpretations and affect the reproducibility of experiments, compelling scientists to adopt strategies to mitigate these obstacles. Awareness of such challenges enhances the quality of data obtained and opens pathways for advancements in the method itself.
Photobleaching and Its Impact
Photobleaching refers to the irreversible loss of fluorescence from a fluorophore due to photochemical reactions induced by the light source. This phenomenon can significantly affect the quantitativeness and precision of measurements. The extent to which photobleaching occurs is largely contingent upon:
- Light Intensity: Higher light intensity can accelerate photobleaching.
- Duration of Exposure: Prolonged exposure can lead to rapid degradation.
- Chemical Environment: The presence of oxygen and other reactive species can hasten the process.
The impact of photobleaching can lead to significant challenges, particularly in long-term studies. Fluorescent signals may diminish, resulting in underestimation of the analyte concentration or erroneous kinetic data in experiments. Consequently, researchers need to employ techniques to minimize photobleaching, such as using less aggressive light sources, applying antifade reagents, or utilizing techniques like time-resolved fluorescence measurements. These approaches may help maintain the integrity of the fluorescent signal over time.
Background Interference
Background interference arises from various sources, affecting the clarity of the fluorescent signal. This interference can originate from scattered light, autofluorescence from the sample matrix, or other emitting materials in the environment. The presence of such extraneous signals complicates data interpretation and may lead to inaccurate conclusions regarding sample characteristics.
Mitigating background interference involves several strategic considerations:
- Proper Sample Preparation: Ensuring that samples are prepared in a manner that minimizes unwanted emissions.
- Wavelength Selection: Utilizing filters to allow only the desired emission wavelengths can help reduce unwanted signals.
- Calibration: Frequent calibration of the instrument helps account for background noise and improves data reliability.
In the realm of fluorescent spectrophotometry, the intricacies of background interference need constant attention to ensure accurate readings and consistent results.
In summary, challenges such as photobleaching and background interference significantly influence the effectiveness of fluorescent spectrophotometry. By acknowledging and addressing these obstacles, researchers can enhance data quality and advance the scope of their investigations in various scientific fields.
Future Directions
The future of fluorescent spectrophotometry holds immense potential for enhancing scientific research and practical applications. As the field evolves, integrating newer technologies and methodologies ensures more efficient and effective outcomes. This section discusses the critical aspects of future directions, emphasizing the integration with other spectroscopic techniques and advancements in automation.
Integration with Other Spectroscopic Techniques
Combining fluorescent spectrophotometry with other spectroscopic methods can substantially increase the analytical capabilities of researchers. Techniques such as mass spectrometry, nuclear magnetic resonance, and infrared spectroscopy can provide complementary data. This integration allows for a more comprehensive analysis of complex samples.
Benefits of Integration:
- Enhanced Sensitivity: Combining methods can improve detection limits and provide more accurate quantification of lower concentrations of analytes.
- Broader Application Scope: Integration facilitates the exploration of new fields, such as proteomics and metabolomics, where understanding molecular interactions is crucial.
- Real-Time Analysis: Techniques like flow cytometry enhance the ability to analyze fluorescent signals in real-time, which is valuable in dynamic biological systems.
There are various ongoing projects that showcase how integrated approaches yield effective results. Researchers are exploring multiplexing techniques, where different spectral data are combined to generate more detailed profiles of samples. Such approaches can lead to breakthroughs in personalized medicine and targeted drug delivery.
Advancements in Automation
Automation is a significant trend that promises to revolutionize fluorescent spectrophotometry. It streamlines processes, reduces human error, and increases throughput in laboratories. Automated systems can handle sample preparation, measurement, and data analysis with minimal operator intervention.
Considerations for Advancements in Automation:
- Scalability: Automated systems allow laboratories to manage larger sample sizes efficiently. This is critical in high-throughput environments such as pharmaceutical testing.
- Data Consistency: Automated measurements minimize variability linked to manual handling, ensuring more reproducible results.
- User-Friendly Interfaces: Modern automation technology often features intuitive software that simplifies operation and data retrieval.
"The integration of automation in fluorescent spectrophotometry is likely to push the boundaries of what is possible, enabling researchers to focus more on interpretation rather than repetitive tasks."
Closure
Fluorescent spectrophotometry is a valuable analytical tool that has made significant contributions to various scientific fields. Its importance lies in its ability to provide real-time insights into molecular interactions, environmental conditions, and biochemical processes. This article highlighted several specific benefits and considerations related to this technique, emphasizing its versatility and relevance in both research and practical Applications.
Summary of Key Insights
Throughout the article, we explored crucial aspects of fluorescent spectrophotometry. The key points can be summarized as follows:
- Foundational Principles: Understanding fluorescence, Stokes shift, and quantum yield is vital for effectively utilizing fluorescent spectrophotometry.
- Instrumentation: Various types of spectrophotometers, such as single beam and portable ones, play a role in the versatility of data collection. The choice of instrument often depends on the specific requirements of the study.
- Applications: The technique is extensively used in biological, environmental, and material sciences. Its role in cellular imaging and pollutant detection showcases its wide-ranging impact.
- Data Analysis Techniques: Proper interpretation of spectral data is critical. Computational methods, including machine learning, are enhancing analytical capabilities.
- Challenges: Issues like photobleaching and background interference can affect results, and they require careful consideration during experiments.
Implications for Future Research
Looking ahead, several implications for future research can be drawn from the advancements and limitations discussed in this article:
- Integration with Other Techniques: Merging fluorescent spectrophotometry with other spectroscopy methods can lead to more comprehensive analyses, allowing for deeper insights into complex systems.
- Automation and Efficiency: Enhanced automation in data collection and analysis will likely increase the reliability and speed of fluorescent spectrophotometry, making it more accessible for various laboratories.
- Development of New Probes: Continued innovation in fluorescent probes will likely improve sensitivity and specificity, thus expanding the range of applications.
- Focus on Environmental Monitoring: As global awareness of environmental issues rises, further research using fluorescent spectrophotometry could aid in monitoring and mitigating pollution.