Proton Rays: Properties, Interactions, and Applications
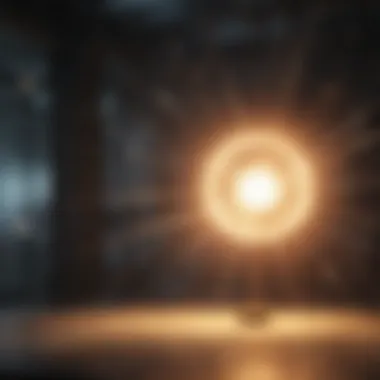
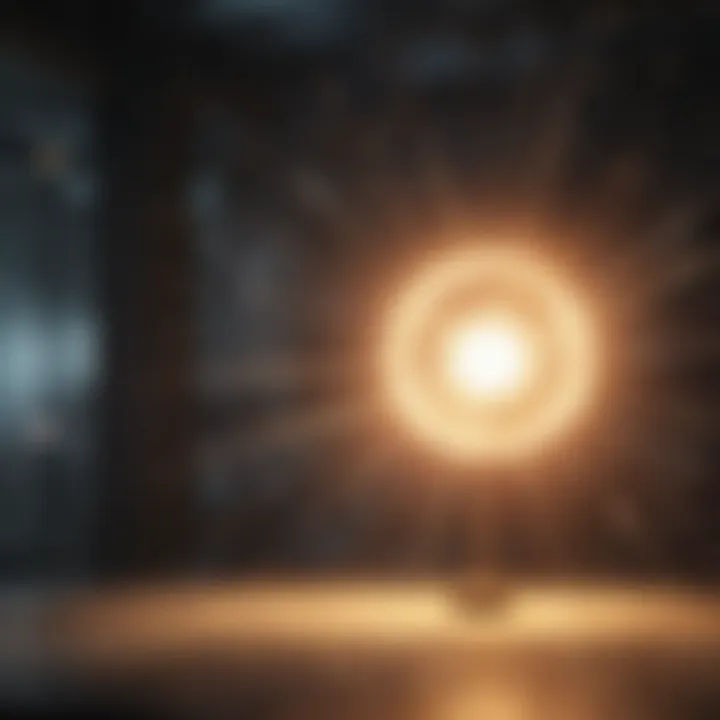
Intro
Proton rays have emerged as a significant area of interest in modern physics and applied sciences, particularly due to their unique properties and wide-ranging applications. These highly energetic charged particles, formed when protons are accelerated to high velocities, play a crucial role in various scientific fields, from medical therapies to nuclear research. Understanding proton rays not only illuminates their fundamental nature but also showcases their potential in advancing technology and enhancing our understanding of the universe.
As we journey through the intricate world of proton rays, we will explore their fundamental characteristics, how they interact with matter, and the cutting-edge applications that harness their power. An appreciation of the historical narrative surrounding proton rays adds context to their current research trajectory, while a look at the innovative techniques for generation and detection reveals the leap science has made in this domain. This comprehensive exploration arms students, researchers, and enthusiasts with the knowledge to appreciate the significant role proton rays play in both theoretical and practical realms.
Research Background
Overview of the Scientific Problem Addressed
Proton rays present a fascinating scientific puzzle. Their behavior when interacting with different materials raises questions relevant across various fields. Scientists seek to understand how these rays can be both influential in energy transfer and potentially harmful if not properly controlled. This dual nature emphasizes the need to study their properties meticulously, especially in contexts such as radiation therapy, where precision is paramount.
Historical Context and Previous Studies
The fascination with protons can be traced back to early 20th century physics. The discovery of the proton itself was a groundbreaking milestone, leading to further investigation into its properties. Researchers like Ernest Rutherford laid the groundwork for understanding atomic particles, setting the stage for future explorations into accelerated protons. As research evolved, the advent of particle accelerators in the 1940s allowed for the first practical applications of proton rays, most notably in medical treatments for cancer, showcasing their potential in the healing arts.
Numerous studies have documented the effectiveness of proton therapy compared to traditional photon-based treatments. The precision targeting of tumors while sparing surrounding healthy tissues makes proton therapy a compelling subject for ongoing research. Investigating these properties fosters continual advancements in therapeutic technology and safety mechanisms, reflecting the historical importance of proton rays.
Findings and Discussion
Key Results of the Research
In the context of modern applications, recent findings underscore the versatility of proton rays. Studies have indicated that their controlled utilization in radiation therapy can significantly reduce side effects when compared to conventional methods. Furthermore, their applicability extends beyond medicine; they facilitate research in particle physics, contributing to a better understanding of fundamental forces and particles in the universe.
Interpretation of the Findings
The ability of proton rays to focus energy on a specific point lends itself to precision treatments. This translates into tangible benefits in patient outcomes, as well as potential advancements in experimental setups for research. By interpreting these results, it's evident that proton rays are not simply novelty; they are a transformative tool that invites further inquiry and refinement. The specificity of proton interaction with matter allows researchers to craft methodologies that maximize effectiveness while mitigating risks.
In summation, this exploration of proton rays delineates their significance in our scientific landscape. By navigating through their properties and applications, we cultivate a deeper understanding of how these energetic particles continue to shape innovations in medicine and technology.
Prologue to Proton Rays
The realm of particle physics invites a rich tapestry of concepts and innovations that shape not only scientific understanding but also practical applications across numerous disciplines. In this vast field, proton rays stand as a fascinating subject of exploration, embodying both fundamental science and technology advancements. Understanding proton rays is crucial because they bridge the gap between theoretical physics and real-world applications, particularly in medical and research domains.
Proton rays are high-energy beams of protons that have a distinct set of characteristics, making them uniquely suited for various applications, from therapies in healthcare to experiments in particle physics. The importance of this introduction lies in several key elements:
- Foundation of Knowledge: Grasping the nature of proton rays allows for a more profound comprehension of advanced topics within particle physics.
- Practical Implications: Insights into proton rays facilitate innovations in fields such as cancer treatment, where proton therapy has emerged as a noteworthy alternative.
- Interdisciplinary Relevance: Learning about the historical development and properties of proton rays paves the way for interdisciplinary dialogues among physicists, engineers, and healthcare professionals.
This section sets the stage by focusing on the definition of proton rays and their defining characteristics, followed by a brief historical context that emphasizes their evolution. This discussion is not merely academic; it serves to highlight why proton rays have garnered significant attention and investment across various sectors.
Definition and Characteristics
Proton rays can be defined as streams of protons—subatomic particles with a positive charge—that travel at high velocities. They are known for their distinct characteristics which include:
- Mass and Charge: Protons have a substantial mass (approximately 1836 times that of electrons) and carry a single positive charge, making them fundamental to the structure of atomic nuclei.
- High-Energy Emissions: When accelerated, these particles can attain significant energies, allowing them to penetrate materials more effectively than many other particle types. This property is particularly useful in applications like proton therapy in medicine.
- Ionization Capability: When interacting with matter, proton rays are efficient at causing ionization, which refers to the process of stripping electrons from atoms. This feature is significant for both therapeutic and experimental purposes.
- Range Versatility: Unlike X-rays, proton rays can be precisely directed to deposit energy at specific depths within a medium, minimizing damage to surrounding healthy tissues during treatments.
These characteristics make proton rays exceptionally valuable in contexts where precision and efficacy are paramount.
Historical Background
The journey of proton rays can be traced back to the early 20th century, specifically following the discovery of the proton by Ernest Rutherford in 1919. Rutherford's experiments laid the groundwork for understanding how these particles could be produced and their potential applications. His pioneering work saw protons as more than just components of atoms—they became a focus of research that would lead to ground-breaking discoveries.
- Advancements in Acceleration: In subsequent decades, innovations such as cyclotrons and synchrotrons emerged, enabling scientists to accelerate protons to high energies. This technological leap was crucial in transforming the theoretical study of particles into practical applications.
- Proton Therapy: The 1950s marked the introduction of proton therapy as a clinical practice, showcasing how proton rays could be deployed effectively in treating cancer. This advancement forever changed the landscape of medical physics, establishing proton therapy as an alternative to traditional radiotherapy methods.
- Continuous Evolution: Over the years, research into proton rays has only expanded, with significant investments made in accelerator technologies and detection methods. As our understanding of their properties deepens, new avenues for application continue to unfold, promising even greater contributions to both science and society.
"Historical milestones in the study of proton rays are not just footnotes in scientific literature; they are the building blocks of modern physics and medical practices."
Overall, the exploration of proton rays is not just about understanding a type of particle; it symbolizes humanity's quest for knowledge and its application—from cosmic discoveries in particle physics to life-saving technologies in medicine.
Fundamental Physics of Proton Rays
Understanding the fundamental physics of proton rays is crucial, as it lays the foundation for various applications across many fields. The core elements, such as their nature and the methods by which they are produced, reveal insights that connect theoretical physics with practical uses. This section delves into these elements, bringing to light the benefits and considerations surrounding proton rays.
Nature of Protons
Protons are positively charged particles found in the nucleus of atoms, playing a significant role in the structure of matter. Their mass is about 1836 times that of an electron, which puts them front and center in nuclear stability and chemical properties.
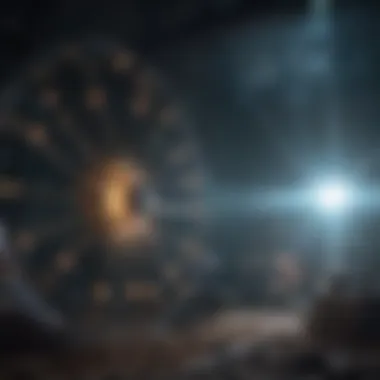
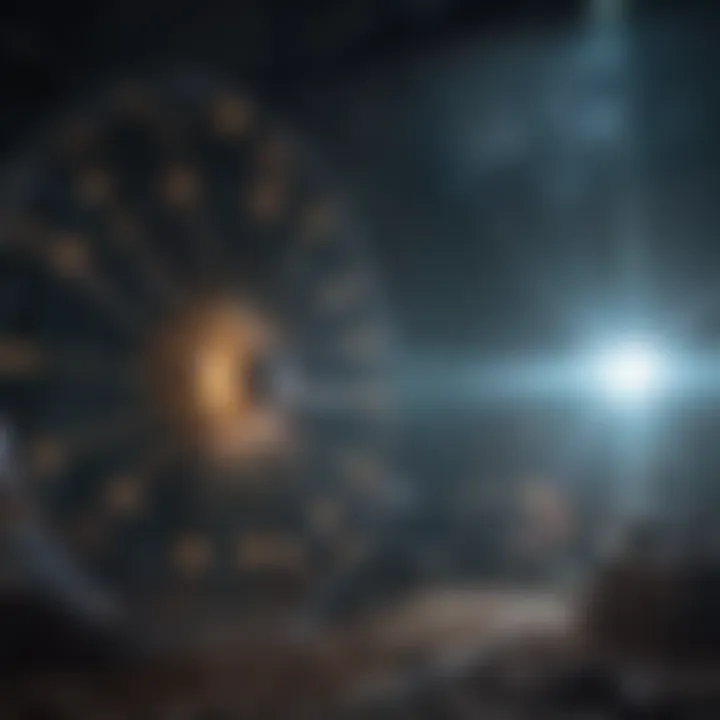
In terms of behavior, protons exhibit strong nuclear forces that keep them bound within the nucleus. Their interactions can be understood through quantum mechanics, which describe their wave-particle duality. For instance, a proton can behave like a particle in some experiments while exhibiting wave-like properties in others. This dual nature is a foundational aspect of their characteristics, which proves essential for the generation and manipulation of proton rays in various applications.
Moreover, protons are critical in nuclear fusion processes, such as those powering the sun. As researchers climb the ladder of understanding in nuclear and particle physics, they uncover ever-deeper layers of how protons function. These insights are not just academic; they pave the way for innovation and discovery across multiple scientific domains.
Production of Proton Rays
Proton rays can be generated through a couple of main avenues, primarily via particle accelerators and cosmic ray interactions. Each method has its unique features and implications.
Accelerators
Particle accelerators are large machines designed to propel charged particles, including protons, at high speeds. The energy levels achieved allow for the creation of proton rays that can be utilized in a range of scientific fields, from fundamental physics to medicine.
A key characteristic of these accelerators is the control they offer. Operators can adjust the energy and intensity of the proton beams to meet specific requirements. This versatility is invaluable in research settings, ensuring that proton rays can be tailored for unique experiments and medical applications, like proton therapy for cancer.
However, accelerators can be quite complex and costly. Their construction and maintenance require significant resources, meaning that not every research facility can afford them. Still, their capability to produce precise, directed proton beams makes them a preferred choice for many scientists.
Cosmic Ray Interactions
Cosmic ray interactions involve high-energy protons and atomic nuclei that bombard the Earth from outer space. These interactions result in a cascade of particles, including secondary proton rays, contributing to our understanding of high-energy physics.
One of the big perks of studying cosmic ray interactions is that they occur naturally, providing a steady stream of data without the need for expensive equipment. Moreover, the unique conditions found in space—as well as the extremely high energy levels—offer a perspective that cannot be replicated in laboratories.
However, observing and utilizing cosmic ray interactions comes with some challenges. The unpredictable nature of cosmic ray events makes it hard to conduct controlled experiments. Scientists often rely on specially designed observatories to detect and analyze these rays, which can limit the frequency and scope of data collection.
In summary, the fundamental physics of proton rays, rooted in the nature of protons and their production methods, forms the backbone of numerous applications across science and medicine. Understanding these components allows for better research and innovative solutions in health, technology, and beyond.
Interaction of Proton Rays with Matter
Understanding how proton rays interact with matter is crucial for unveiling their vast applications, particularly in fields like medicine and particle physics. The interaction can be viewed through various lenses, including the ways in which these rays transfer energy, their ability to ionize surrounding atoms, and the resultant biological and material effects. This comprehension shapes not just scientific inquiry but also practical applications.
Ionization Processes
When proton rays traverse through matter, they collide with atoms, resulting in ionization. This process is pivotal for various applications, especially in radiation therapy for cancer patients. In radiation therapy, protons are aimed very precisely at tumors. As they strike atoms within the target tissue, they can knock electrons free, producing ions. This ionization can disrupt atomic structures, leading to cell damage or destruction, which is ultimately useful in targeting cancer cells while sparing healthy tissue.
The degree of ionization that occurs depends on the energy of the protons. For instance, protons with high energy can travel further in tissues, resulting in a broader range of ionization events. Here is a simplified look at the interaction:
- Energy Transfer: Higher energy allows deeper penetration.
- Localized Effect: Protons can be targeted to minimize damage to surrounding tissues.
In medical applications, understanding these ionization processes helps physicists design treatment plans that maximize therapeutic benefits.
Energy Loss Mechanisms
Energy loss is a significant aspect when protons pass through materials. This occurs primarily through two mechanisms: ionization and excitation of atoms. Essentially, as protons pass through matter, they lose energy in predictable patterns.
An interesting feature of energy loss in proton interactions is the Bragg peak phenomenon. This refers to the sharp increase in energy deposited just before the protons come to a stop in the targeted tissue, allowing for effective treatment while minimizing damage to adjacent cells. The factors affecting energy loss include:
- Material Density: Denser materials lead to greater energy loss.
- Charge Interaction: Positively charged protons interact with negatively charged electrons and atoms in the material causing energy changes.
Understanding these energy loss mechanisms is critical not only for developing efficient therapies but also for optimizing detectors in research setups, effectively tracking how protons behave in experimental conditions.
Radiation Effects
The radiation effects caused by proton interactions with matter can be classified into two main categories: direct and indirect effects. The direct effects occur when the proton directly ionizes atoms and molecules within the target. This can lead to cellular damage. In contrast, indirect effects stem from secondary radiation, such as free radicals generated during ionization.
These effects must be understood holistically:
- Cellular Damage: Ionization can lead to mutations, impacting cellular function.
- Biological Response: The body can sometimes repair minor damages, yet significant exposure can lead to severe consequences.
"The safety and efficacy of proton therapy depend on a deep understanding of the intricate interplay between proton rays and biological tissues."
The implications of radiation effects extend to both therapeutic practices and safety regulations, emphasizing the importance of meticulous planning in any experimental or medical use of proton rays.
Detection Techniques for Proton Rays
Understanding the detection techniques for proton rays is pivotal, not just for effective measurements but also for advancing research and applications of proton beams. The methodologies employed in detecting these rays have evolved significantly over the decades. This section focuses on the historical methods that laid the groundwork and the modern technologies that enhance accuracy and efficiency in detection. Each approach has its merits and drawbacks while contributing to the multifaceted landscape of proton ray science.
Historical Detection Methods
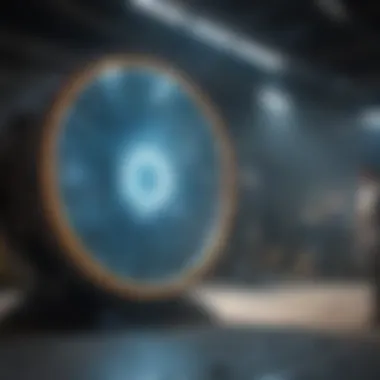
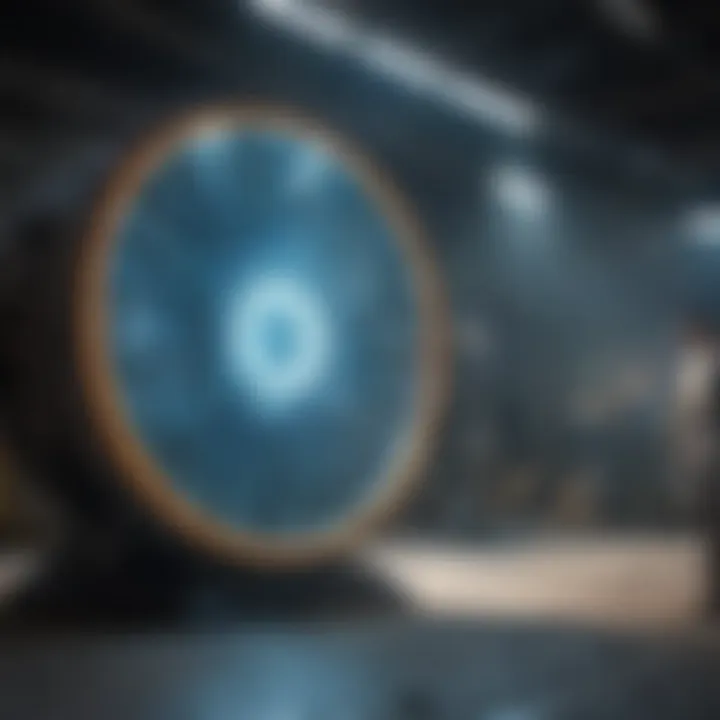
In the early days, the detection of proton rays was a complex endeavor, often employing rudimentary techniques. Historically, the classic cloud chamber was a primary tool for observing charged particles, including protons. This device allowed for visualizing the paths of ionized particles as they interacted with supersaturated vapor, producing trails that physicists could analyze.
Another notable method was the photographic plate, which could capture and record the scattering of proton beams. Photographic emulsions were particularly popular in the mid-20th century for research in cosmic rays, exhibiting the ability to store energy deposited by proton interactions, albeit with a need for time-intensive development and analysis.
Over time, these historical methods laid the foundation for more refined techniques. They highlighted the necessity for improved precision and efficiency, resulting in innovations that would shape the future of particle detection.
Modern Technology in Detection
Solid-State Detectors
Solid-state detectors represent a significant leap forward in the detection of proton rays. These detectors utilize semiconductor technology to convert incoming particles into electrical signals. The primary characteristic of solid-state detectors is their compact size and high sensitivity, making them a versatile choice in both research and medical applications. The unique feature of these detectors is their ability to achieve high spatial resolution, which is essential for mapping proton interactions accurately.
Advantages of solid-state detectors include:
- Rapid response time, allowing for real-time data collection.
- Reduced intervention needed for calibration compared to older methods.
- Capability for integration with advanced digital readout systems for enhanced analysis.
However, one disadvantage is their susceptibility to radiation damage over prolonged use, which can affect long-term accuracy.
Gas Detectors
Gas detectors feature prominently in the detection of proton rays, especially in high-energy physics experiments. These detectors operate by detecting ionization produced when protons collide with gas molecules. They are often characterized by their ability to measure energy and charge simultaneously, making them a robust tool in various applications.
One unique advantage of gas detectors is their ability to function across a wide range of energies, accommodating different experiments seamlessly. Additionally, they offer:
- Flexibility in terms of the types of gases used, optimizing detection based on specific needs.
- The capacity to handle larger volumes than solid-state detectors, which can be beneficial in experiments requiring extensive interaction zones.
On the flip side, gas detectors generally require more extensive setup and calibration, increasing the complexity of the experimentation process. Their sensitivity to environmental factors can also lead to variations in data accuracy.
Medical Applications of Proton Rays
The realm of medicine has consistently sought innovative methods to enhance treatment efficacy while minimizing side effects. Proton rays, a common tool in modern radiotherapy, have emerged as a critical component in the fight against cancer. Their unique properties allow for precision that traditional X-ray therapies often struggle to achieve. This section delves into two vital aspects of medical applications: proton therapy and dosimetry in medical physics.
Proton Therapy
Proton therapy is a type of particle therapy that utilizes high-energy protons to target cancerous tissues. The notable feature here is the Bragg Peak; this unique phenomenon occurs when protons deposit most of their energy at a specific depth within the tissue. Unlike photons from traditional radiation, which irradiate not only the target tumor but also healthy surrounding tissue, proton rays can be finely tuned. This tailored approach reduces collateral damage to healthy cells, leading to fewer side effects—an advantage that is particularly beneficial in treating pediatric patients or tumors near critical organs.
The practical applications of proton therapy have shown promising results. For example, patients with tumors in the brain, spine, or head and neck regions have reported fewer complications and improved quality of life post-treatment. Proton therapy is likewise effective in treating solid tumors, such as those found in the breast or lung, where precision is paramount. The ability to meticulously direct protons allows oncologists to achieve significant doses at the tumor site while sparing healthy tissue, aligning with the overall goals of oncological treatment.
However, establishing a proton therapy center involves considerable financial investment and logistical challenges. Many institutions leveraging this approach find themselves in a competitive academic landscape, trying to prove the clinical benefits over established modalities. Yet, as the data accumulates, the demand for proton therapy is likely to rise, transcending barriers both technical and financial.
Dosimetry in Medical Physics
Dosimetry refers to the measurement and calculation of the doses delivered to patients during radiation therapy. It's an essential component in ensuring that treatments maximize tumor control while minimizing exposure to surrounding healthy tissue. With proton therapy, accurate dosimetry is critical due to the need to calculate the precise range of protons in tissues, which can vary widely based on multiple factors, such as the patient's anatomy and the density of the tumor.
Key considerations in dosimetry include:
- Calibration of equipment: Ensuring that the equipment used for proton delivery is accurately calibrated to measure the doses delivered.
- Patient-specific planning: Developing treatment plans based on individual patient anatomy, utilizing advanced imaging techniques to visualize the tumor and healthy surrounding tissue.
- Monitoring delivery: Real-time monitoring systems are crucial to ensure that the treatment adheres to the initial plan, modifying the delivery dynamically if the patient's position changes or if any other factors arise during the treatment session.
"In proton therapy, dosimetry is not just about calculating doses; it’s about ensuring that every proton delivered serves its purpose in fighting cancer."
Thus, dosimetry in proton therapy can be seen as both an art and a science—a field where precision meets patient care, guiding medical physicists in their mission to deliver optimal treatment results. The integration of advanced software and modern techniques has opened doors for innovation in this area, promising to enhance accuracy and safety in clinical settings.
In summary, the medical applications of proton rays are vast and transformative. Proton therapy stands out as a beacon of hope for patients needing precise, effective cancer treatment, while sophisticated dosimetry practices ensure the safe and accurate application of this promising technology.
Proton Rays in Research
Proton rays serve as a cornerstone in various fields of research, illuminating paths that were previously shrouded in mystery. Their unique properties make them invaluable in experiments aimed at understanding the fundamental components of matter and the forces that govern them. In this section, we explore the specific roles that proton rays play in particle physics and material science, two areas where their implications can shape future developments in technology and theoretical physics.
Applications in Particle Physics
In particle physics, proton rays have created ripples through the scientific community, primarily due to their ability to provide insight into the subatomic world. One of the crucial applications is in high-energy particle accelerators, like the Large Hadron Collider (LHC). These facilities use proton collisions to explore fundamental questions regarding the composition of matter at a very small scale. When protons collide at near-light speed, the resulting interactions can lead to the creation of new particles, some of which have never been observed before.
The collision data garnered from these experiments contribute significantly to our understanding of the Standard Model of particle physics. Proton rays also facilitate investigations into deeper questions about dark matter and energy, two enigmas that continue to perplex scientists today. The unique interaction cross-sections of protons allow physicists to probe into realms where phenomena such as supersymmetry or extra dimensions might exist.
Key benefits of utilizing proton rays in particle physics include:
- Precision Measurement: Their well-understood nature helps in obtaining accurate measurements of fundamental forces.
- Particle Creation: Collisions produce various secondary particles, leading to insights about their interactions.
- Histogram Techniques: Data analysis methods, including histograms, aid in categorizing and quantifying results from experiments.
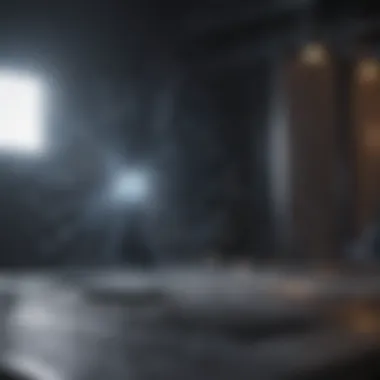
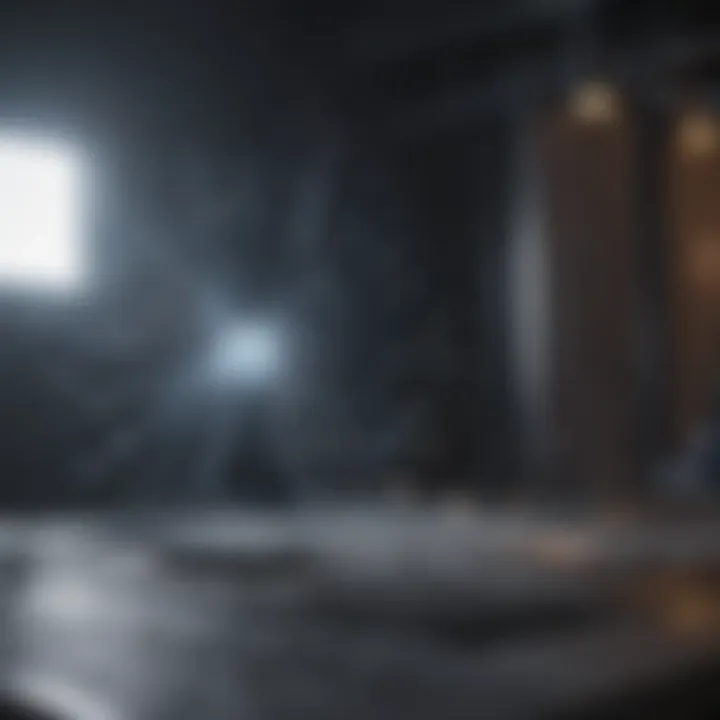
"The study of proton interactions opens the door to the microscopic universe where mystery reigns supreme."
Uses in Material Science
Beyond particle physics, proton rays find essential applications in material science, particularly in the analysis of materials at the atomic level. Techniques such as Proton Induced X-ray Emission (PIXE) capitalize on proton interactions to identify and quantify elements within materials. This is especially useful in fields such as archaeology, art, and environmental science, where understanding material composition is crucial.
In material science, proton rays enhance our ability to:
- Modify Material Properties: Proton beams can induce changes at the molecular level, making materials stronger or more resistant to certain conditions.
- Characterize Materials: Researchers can determine properties such as composition, structure, and defects in solids through targeted proton bombardment.
- Nanotechnology: Proton rays are employed in the fabrication of nanoscale devices, expanding capabilities in electronics and medicine.
The adaptability of proton rays in research is a testament to their potential. As we refine our experimental methods and develop better technologies for using these rays, we may unlock further innovations applicable across different scientific domains.
Safety Considerations
Ensuring safety when working with proton rays is paramount, as improper handling can lead to significant health risks and environmental concerns. The nature of proton rays, particularly their ability to ionize atoms and molecules, emphasizes the need for prudent practices in both research and clinical settings. Understanding the potential hazards associated with proton therapy, radiation exposure, and particle physics experiments promotes an environment where scientific exploration can proceed without undue risk.
Radiation Protection Guidelines
Radiation protection guidelines serve as the cornerstone of safety measures in environments involving proton rays. These guidelines are crafted to minimize exposure and enhance worker and patient safety. Important elements include:
- Distance: Increase the distance between the source of radiation and the individual. This basic principle plays a vital role in reducing exposure levels.
- Shielding: Use materials that effectively absorb or block proton rays. Common substances involved in shielding include concrete, lead, and specialized polymers. These materials can prevent or mitigate the harmful effects of radiation on those nearby.
- Time Management: Limit the duration of exposure. Reducing the time spent near radiation sources dramatically decreases the potential for harmful effects.
- Monitoring: Regular monitoring of radiation levels in both medical and research settings helps maintain safe conditions. Personal dosimeters and area monitors ensure compliance with established safety standards.
"Safe practices and compliance with radiation standards not only protect lives but also enhance the quality of research and therapy."
Implementing these guidelines ensures that the benefits of proton therapy and related research outweigh the risks, creating a safer space for innovation and healing.
Risk Assessment Strategies
Effective risk assessment strategies further bolster safety in environments with proton rays. These strategies focus on identifying potential hazards and implementing preventative measures. Key approaches can include:
- Hazard Identification: Evaluate all operational practices involving proton rays to identify possible safety hazards. This could encompass equipment malfunctions, improper handling procedures, and environmental factors that may elevate risk.
- Risk Analysis: After identifying hazards, analyze their potential consequences. This analysis often considers severity and likelihood, allowing for a better understanding of areas requiring increased safety measures.
- Implement Control Measures: Once risks are assessed, it's crucial to implement control measures. This may involve investing in better shielding materials, refining operating procedures, or enhancing staff training programs.
- Continuous Feedback Loop: Upkeep involves regular reviews and updates to safety protocols. Consistent feedback from workers and researchers can reveal new hazards or inefficiencies in existing strategies, ensuring that risk management evolves alongside advancements in technology and practice.
Effective risk assessment is not merely about mitigating danger; it fosters a culture of safety that encourages innovation while prioritizing human welfare. In this regard, ongoing education and training for both professionals and students are essential in creating an informed workforce that respects the power of proton rays.
Future Directions in Proton Ray Research
As science continues to advance at a breakneck pace, the realm of proton ray research is no exception. The growing significance of proton rays in various fields implies a pressing need to explore new technologies and applications. Future directions in this area represent a tantalizing opportunity for both researchers and industries, spotlighting the potential that proton rays hold in tackling complex challenges in healthcare, materials science, and fundamental physics.
Advancements in Accelerator Technology
The backbone of proton ray research lies in the advancement of accelerator technology, which has made remarkable progress over recent years. Proton accelerators, once cumbersome behemoths, are transforming into more compact systems.
- Miniaturization: With novel designs such as cyclotrons and synchrotrons, researchers are developing smaller, yet more efficient accelerators. These advancements can open doors to widespread applications, making installations feasible in hospitals and research institutes lacking enormous space.
- Cost Efficiency: Alongside compact designs, the cost of operating these accelerators is decreasing. This is largely due to better materials and more efficient methods that reduce energy consumption. For instance, superconducting magnets require less power while providing greater performance, pushing feasibility for even small labs to participate in pioneering research.
- Enhanced Precision: The ability to produce beams of protons with tighter energy spreads and improved controllability broadens horizons in precision therapy, especially in medical applications. Proton therapy, which uses targeted proton beams to treat cancer, can gain benefits from advancements in accelerator technology.
Emerging Applications
Proton rays are paving the way for numerous emerging applications that promise to reshape scientific and medical landscapes. Here’s a closer look at some notable areas of exploration:
- Nanomedicine: Researchers are investigating how proton beams interact with nanoscale systems to enhance drug delivery mechanisms. The ability to target a drug with pinpoint accuracy could revolutionize cancer treatment, decreasing damage to surrounding healthy tissue.
- Material Aging Studies: Proton rays are increasingly used in assessing the aging processes of materials. In industries like aerospace or nuclear power, understanding how materials degrade under radiation can lead to safer and longer-lasting components.
"The future is not a gift; it's an achievement." - Robert F. Kennedy
- Space Exploration: With an uptick in missions exploring Mars and beyond, proton rays help scientists understand cosmic radiation's impact on astronauts and technology in space. This knowledge is essential for ensuring the safety and functionality of interplanetary endeavors.
- Quantum Computing: As quantum technology advances, proton beams can play a critical role in examining quantum structures. Understanding how proton interactions affect quantum bits may lead to breakthroughs in computing capabilities.
The future of proton ray research shines brightly, driven by promising advancements in both technology and applications. This not only enhances our understanding of the universe but also improves our daily lives through significant medical and industrial breakthroughs.
Epilogue
In this exploration of proton rays, we laid down the essential dynamics and nuances surrounding these particles, giving readers a keen understanding of why they matter. The significance of the topic cannot be overstated, particularly within the realms of physics, medicine, and industry.
Summary of Key Points
Proton rays, as a subject of study, encapsulate several critical areas:
- Nature and Characteristics: We examined their fundamental properties, including mass, charge, and behavior under various conditions.
- Production Methods: The discussion highlighted techniques such as particle accelerators that enable the generation of proton rays.
- Interaction Mechanisms: Proton rays offer unique insights into their interaction with matter— from ionization processes to energy loss mechanisms.
- Detection Technologies: Historical and state-of-the-art detection methods were reviewed, showcasing how technology keeps advancing to measure these rays effectively.
- Medical Applications: The medical field benefits vastly from proton therapy, a targeted treatment option that minimizes damage to healthy tissue, which was underscored with practical implications and dosimetry considerations.
- Future Directions: The article underscored the promising advancements in accelerator technology and emerging applications in research that invoke a sense of anticipation and excitement.
Final Thoughts
In summation, the discourse on proton rays posits them as not just mere particles but pivotal elements in understanding and utilizing physical laws for better applications. Their multifaceted nature opens doors in numerous fields, from groundbreaking cancer treatments to pioneering research in particle physics. Ensuring a solid grasp of this topic is crucial not only for researchers but also for educators and students alike as they navigate the intricate web of scientific inquiry. As we peep into the future, the evolving landscape of proton ray applications is likely to unveil even more profound impacts on science and technology.
"Understanding the dynamics of proton rays is like peering into the very nature of the universe itself."
Keeping informed on advancements and ongoing research in this area can illuminate pathways for the next generation of scientists and innovators.