Understanding X-Ray Diffraction Spectroscopy
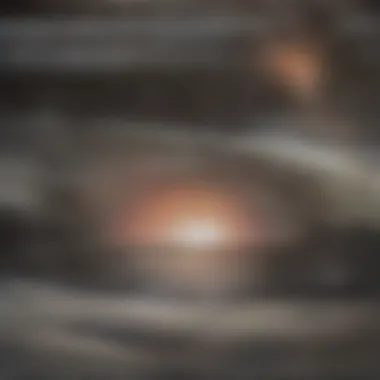
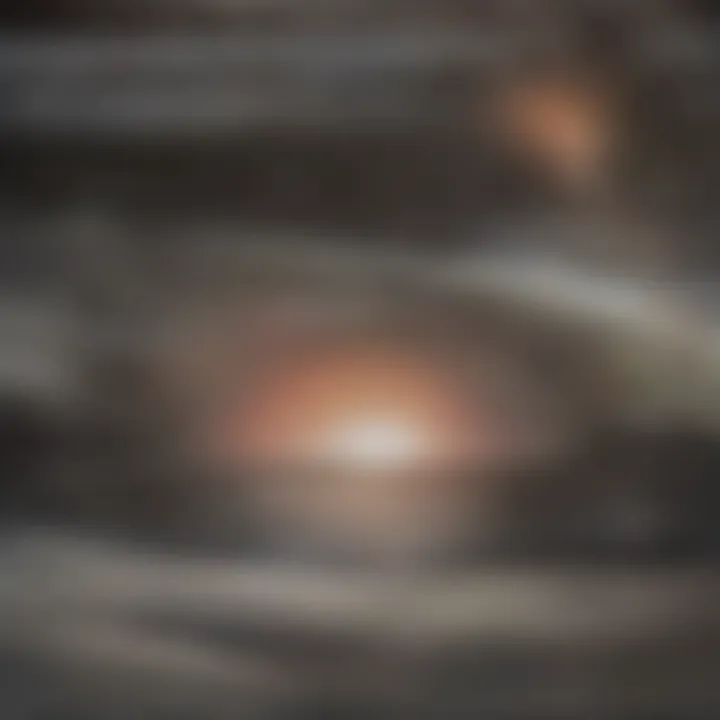
Intro
X-ray diffraction spectroscopy is a powerful technique used extensively in various fields of science and engineering. It allows researchers to analyze material structures at the atomic level. The intricacies of how X-rays interact with matter provides deep insights into the properties of different materials. Understanding this technique is fundamental not just for academic researchers and professionals, but also for students who are engaged in material sciences, biology, and chemistry.
In this article, we will explore the foundational principles of X-ray diffraction spectroscopy and its diverse applications. We will also discuss important historical developments that have shaped this practice, along with modern advancements that continue to enhance its utility in scientific research.
Research Background
Overview of the Scientific Problem Addressed
The study of crystal structures is essential in various scientific disciplines. X-ray diffraction spectroscopy assists in solving the critical problems regarding structural determination of crystalline materials. The arrangement of atoms within a crystal significantly influences its properties, affecting physical and chemical behaviors. Until the advent of X-ray diffraction, unveiling such structural details remained a challenge for scientists.
Historical Context and Previous Studies
The basis of X-ray diffraction began with the discovery of X-rays by Wilhelm Conrad RΓΆntgen in 1895. Shortly thereafter, in 1912, Max von Laue first demonstrated the diffraction of X-rays by crystals. This marked the beginning of an era of atomic level material characterization. Following von Laue, the development of Bragg's Law by William Lawrence Bragg and his father, William Henry Bragg, provided a definitive relationship between the wavelengths of X-rays and the spacing between atomic planes in crystals. Their work laid the foundation for crystallography, creating a pathway for numerous studies that explored materials ranging from simple salts to complex biomolecules.
As research progressed, techniques such as powder X-ray diffraction and single-crystal X-ray diffraction became standard tools. These advancements broadened applications to alloys, ceramics, and polymers among many others, providing invaluable data for materials science, geology, and even biology.
In the recent phase, continuous improvements in X-ray sources and detection technologies have resulted in higher resolution and faster data collection, enhancing the capabilities for structural analysis.
Findings and Discussion
Key Results of the Research
X-ray diffraction spectroscopy has yielded significant results in understanding various material properties. For instance, researchers have successfully characterized the crystal structure of numerous organic and inorganic compounds.
- The identification of specific crystal symmetries helps in understanding material behavior under stress.
- Studies in materials science reveal how subtle changes in crystal structures can lead to vastly different physical properties, an aspect vital in semiconductor development.
Interpretation of the Findings
The implications of these findings are profound. Knowledge derived from X-ray diffraction enables the design and optimization of materials for specific applications. For example, in pharmaceuticals, understanding the crystallographic properties of drugs aids in enhancing their efficacy.
The comprehension of X-ray diffraction techniques also supports advancements in nanotechnology. As materials become more complex and smaller in scale, precise measurement of structural elements at the atomic level is critical for successful innovation.
These findings indicate the significance of X-ray diffraction spectroscopy, not merely as a tool for identification, but also as a cornerstone in developing new technologies and materials.
In sum, this article aims to unravel the complexities of X-ray diffraction spectroscopy, elucidating its role in various scientific inquiries and broadening the understanding of its principles and applications.
Prelude to X-Ray Diffraction Spectroscopy
X-ray diffraction spectroscopy is a significant technique used in various scientific fields, including materials science, chemistry, and biology. It enables researchers to determine the crystalline structure of materials at an atomic level. Understanding this technique is crucial for a detailed analysis of material properties, phase identification, and the investigation of molecular structures. This section aims to provide insight into the foundation of X-ray diffraction spectroscopy, including fundamental definitions, historical contexts, and its relevance today.
Definition and Overview
X-ray diffraction spectroscopy is a tool that utilizes X-rays to explore the structural arrangement of atoms within a material. When X-rays hit a crystalline sample, they are scattered in various directions due to the orderly array of atoms. This scattering leads to the generation of a diffraction pattern, which can be analyzed to obtain information about the crystal structure.
The process involves a simple principle: the spacing of atoms in a crystal lattice corresponds to specific wavelengths of X-rays. By measuring angles and intensities of the scattered X-rays, one can deduce the arrangement of atoms in the crystal, as described by Braggβs Law.
The ability to analyze different material typesβmetals, ceramics, polymers, and moreβmakes this technique versatile. It is not just about finding the structure but also understanding how different factors impact material properties, which is crucial in development and applications of new materials.
Historical Background
The roots of X-ray diffraction date back to the early 20th century. Wilhelm RΓΆntgen first discovered X-rays in 1895, marking a pivotal moment in physics and materials science. The application of X-rays to study crystal structures began shortly thereafter. In 1912, Max von Laue formulated the theory of X-ray diffraction as a method for investigating the atomic structure of crystals.
The subsequent contribution of William Henry Bragg and his son William Lawrence Bragg led to the development of the Bragg's Law, which is essential to interpreting diffraction patterns. Their work earned them the Nobel Prize in Physics in 1915, solidifying the method's significance in crystallography.
Since then, X-ray diffraction has evolved, becoming an indispensable technique in laboratories worldwide for structural analysis. The integration of computer technology in data analysis has further enhanced its capabilities, allowing for precise and complex evaluations of crystal structures.
"The discovery of X-ray diffraction has revolutionized our understanding of matter at the atomic level, paving the way for advancements in various scientific domains."
Fundamental Principles
X-ray diffraction spectroscopy lies at the core of material characterization and analysis. This section elucidates the fundamental principles that govern X-ray diffraction, providing essential knowledge for various applications. Understanding these principles is crucial for researchers to effectively apply the technique in their studies.
X-Ray Production
X-rays are produced when high-energy electrons collide with a target material, typically composed of metals like tungsten or molybdenum. The interaction results in two primary phenomena: characteristic radiation and Bremsstrahlung radiation.
- Characteristic Radiation: This occurs when electrons knock inner-shell electrons out of the target atoms. The subsequent transition of outer-shell electrons into the lower energy states emits X-rays with specific energies dependent on the target element.
- Bremsstrahlung Radiation: This form arises from the deceleration of electrons as they pass near atomic nuclei, emitting a continuous spectrum of X-ray energies.
Both types of X-ray production are vital for generating the radiation needed for diffraction studies, forming the basis of X-ray diffraction spectroscopy.
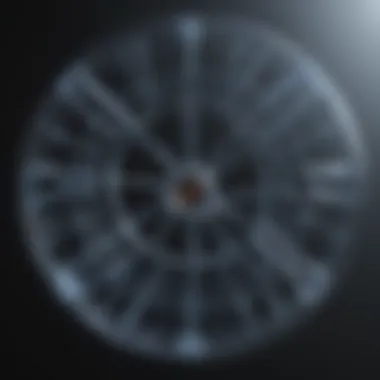
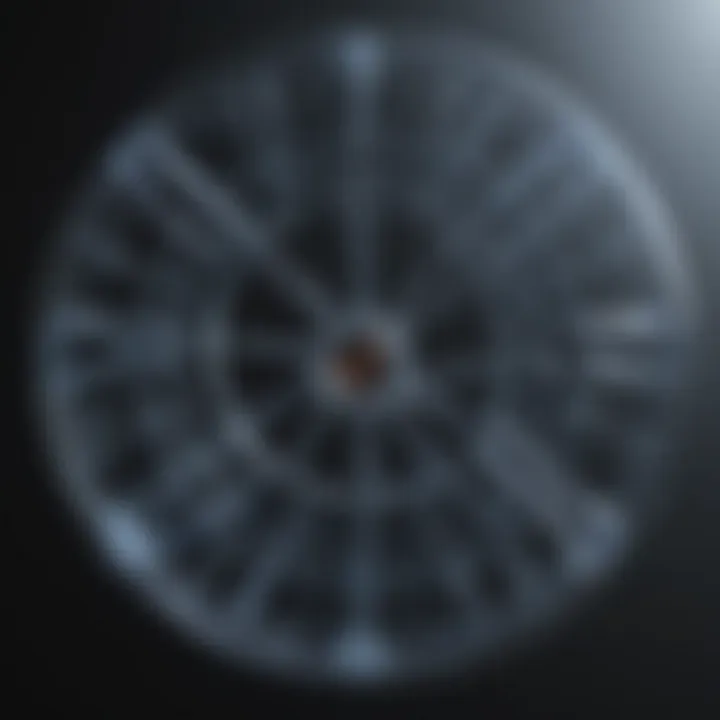
Interaction of X-Rays with Matter
When X-rays encounter a crystalline material, they interact through two primary mechanisms: elastic and inelastic scattering.
- Elastic Scattering: In this process, the energy of the incident X-rays remains unchanged. Instead, the direction of the X-rays is altered upon collision with atoms in the crystal lattice. This change in direction is what is measured in diffraction experiments.
- Inelastic Scattering: Here, some energy is exchanged between the X-rays and the atoms, leading to a change in energy of the scattered X-rays. Although less significant for diffraction patterns, this can provide additional information about the material's electronic states.
The interplay between these interactions and the crystal lattice plays a pivotal role in understanding material properties, making it essential to grasp for effective analysis.
Bragg's Law
Bragg's Law is a foundational principle of X-ray diffraction, providing a mathematical relationship essential for determining the arrangement of atoms within a crystalline structure. The law states:
nΞ» = 2d sin(ΞΈ)
In this equation:
- n is an integer representing the order of the reflected wave.
- Ξ» is the wavelength of the incident X-rays.
- d is the distance between crystal planes.
- ΞΈ is the angle of incidence at which constructive interference occurs.
This relationship indicates that constructive interference in X-ray diffraction patterns occurs when the path difference between reflected rays from adjacent crystal planes is an integer multiple of the wavelength. Understanding this principle allows researchers to extract critical information about the crystal structure, including interplanar spacing and symmetry, which is pivotal in fields such as materials science and chemistry.
Instrumentation in X-Ray Diffraction Spectroscopy
Instrumentation is critical for the accurate and reliable execution of X-Ray Diffraction Spectroscopy (XRD). The equipment used in XRD facilitates the generation, detection, and analysis of X-rays, which are essential to determine the crystal structures and properties of materials. Each component plays a unique role in acquiring high-quality diffraction data, impacting the quality of results for various applications across scientific domains.
X-Ray Sources
X-Ray sources are fundamental to X-ray diffraction experiments. They generate the X-rays needed to probe the sample. Commonly used sources include X-Ray tubes and synchrotron sources. X-Ray tubes produce X-rays when high-energy electrons collide with a target, like Copper or Molybdenum. This method is more accessible and is widely employed in laboratories.
Key aspects of X-Ray sources include:
- Type of radiation emitted: Different target materials result in different wavelengths of X-rays. This can affect the resolution of the measurements.
- Intensity and stability: Higher intensity and stable output are crucial for detecting low-concentration samples.
- Operational cost: Laboratory systems tend to be less expensive than synchrotron facilities, which require vast resources and expertise.
Detectors in XRD
Detectors are designed to capture the diffracted X-rays after they interact with the sample. The choice of detector affects the overall efficiency and accuracy of XRD measurements. Common types include scintillation detectors, semiconductor detectors, and area detectors.
Considerations for X-Ray detectors:
- Sensitivity: The ability to detect low-intensity signals enhances measurement precision.
- Dynamic range: A larger dynamic range allows the detector to accurately measure both weak and strong signals.
- Time resolution: Faster detectors are needed for time-resolved studies, which are increasingly prevalent in research.
"The quality of the detector directly influences data integrity and the conclusions drawn from XRD studies."
Goniometers
Goniometers are devices used to accurately measure the angle of the diffracted rays. They allow for precise positioning of both the sample and the detector relative to the incoming X-ray beam. There are various types of goniometers, including laboratory goniometers and those found in synchrotron facilities.
Importance of Goniometers:
- Accuracy of angles: Precise angular measurements are critical to make valid determinations using Bragg's Law.
- Type of motion: Different goniometers can offer 2ΞΈ or ΞΈ-ΞΈ measurements, affecting how data can be collected.
- Ease of use: Modern goniometers may incorporate automated features, streamlining the operation and reducing user error.
In summary, understanding the instruments utilized in X-ray diffraction spectroscopy provides insight into the system's capabilities and limitations. Properly chosen and maintained equipment leads to better results, enabling researchers to explore diverse fields such as materials science, chemistry, and biology.
Types of X-Ray Diffraction Techniques
The field of X-ray diffraction spectroscopy encompasses a number of techniques that serve different purposes and material types. Each technique offers unique insights into the structural properties of materials, making them crucial for researchers across various disciplines. Understanding these techniques allows scientists to select the most appropriate method based on their specific requirements. Here we discuss four key techniques: Powder X-Ray Diffraction, Single-Crystal X-Ray Diffraction, High-Resolution X-Ray Diffraction, and Small-Angle X-Ray Scattering.
Powder X-Ray Diffraction
Powder X-Ray Diffraction (PXRD) is one of the most widely used techniques in materials characterization. Its main advantage lies in its ability to analyze polycrystalline materials. Typically, a powdered sample is placed in the path of the X-ray beam. As the X-rays interact with the crystal lattice, they produce diffraction patterns that can be recorded and analyzed.
This technique is essential for identifying and quantifying phases present in a sample. Furthermore, PXRD can help in determining crystal structures and sizes, which is invaluable in quality control in industries such as pharmaceuticals and materials science. It is also a straightforward and cost-effective method, making it accessible for many laboratories.
Single-Crystal X-Ray Diffraction
Single-Crystal X-Ray Diffraction (SCXRD) offers a more detailed analysis compared to PXRD. As the name suggests, this technique requires a single crystal of the material being studied. The resulting data provide precise information about the arrangement of atoms within the crystal lattice.
SCXRD is particularly important in fields such as organic chemistry and mineralogy. By resolving complex molecular arrangements, this technique aids in the understanding of molecular interactions and properties. However, it often requires significant sample preparation and may face challenges if the crystal structure is not suitable for analysis.
High-Resolution X-Ray Diffraction
High-Resolution X-Ray Diffraction (HRXRD) is employed to study thin films and epitaxial layers. HRXRD provides a detailed examination of the structural integrity and quality of crystal layers. It is particularly useful in semiconductor research, where the quality of layers can greatly affect electronic properties.
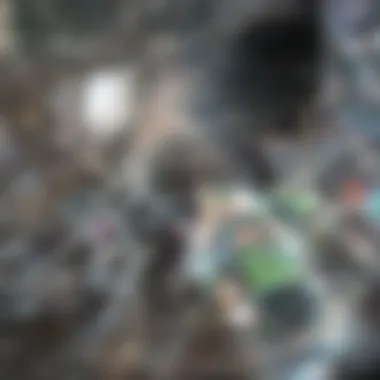
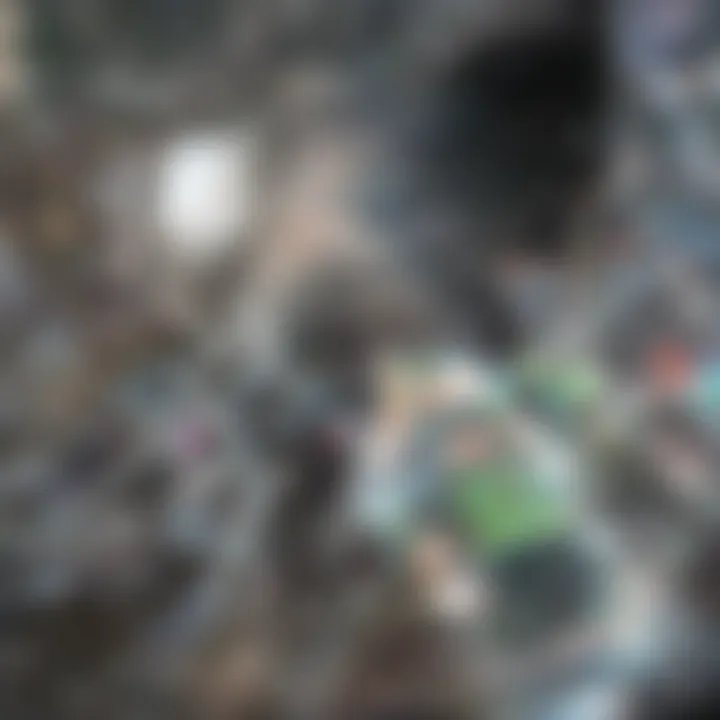
The technique allows for the detection of small variations in lattice parameters and can reveal defects within the crystal structure. As a result, HRXRD is critical for optimizing material properties in advanced technological applications. The information gained from HRXRD can help improve the performance of electronic and optoelectronic devices.
Small-Angle X-Ray Scattering
Small-Angle X-Ray Scattering (SAXS) focuses on the scattering of X-rays at small angles, making it ideal for investigating nanostructured materials. This technique provides information about the shape, size, and distribution of particles in the range of nanometers to micrometers.
SAXS is employed in various fields, including biology, materials science, and nanotechnology. For instance, it can be used to study proteins and polymers, offering insights into their structural organization. Its non-destructive nature is a significant advantage, allowing scientists to analyze samples in their native state.
In summary, understanding the different types of X-Ray Diffraction techniques is vital for selecting the appropriate method to acquire detailed structural information necessary for advancing scientific knowledge.
Data Analysis in X-Ray Diffraction Spectroscopy
Data analysis plays a critical role in X-ray diffraction spectroscopy. It allows researchers to interpret the data collected from experiments effectively. The quality of the analysis can significantly impact the conclusions drawn about a material's structure and properties. As such, understanding the various aspects of data analysis is essential for anyone working in this field.
One of the main benefits of data analysis is that it provides a systematic approach to extract meaningful information from complex datasets. Analyzing data from X-ray diffraction (XRD) experiments helps identify crystalline phases, determine structural parameters, and assess material quality. Here are some key aspects to consider:
- Accuracy: Precise data analysis ensures reliable results, which are crucial for validating experimental hypotheses.
- Methodology: Different techniques exist for analyzing XRD data. Choosing the right method can enhance the quality of results and insights.
- Software tools: Numerous software packages are available for data analysis, simplifying the process of peak identification and structural determination.
The next sections will explore specific methods of data analysis in X-ray diffraction spectroscopy, focusing on peak identification and fitting, structure determination methods, and the Rietveld refinement technique.
Peak Identification and Fitting
Peak identification is one of the first steps in the data analysis process. Each peak in an XRD pattern correlates with a specific crystallographic plane, according to Bragg's Law. Accurate identification of these peaks is essential for further analysis. The main tasks involved are:
- Locating peaks: Using software tools helps automate the peak detection process. Algorithms can analyze the intensity and position of peaks to provide quantitative data.
- Fitting peaks: Once identified, peaks must be fitted to determine their position and shape. Various functions, such as Gaussian or Lorentzian, are used in fitting. This process aids in resolving overlapping peaks.
- Interpreting results: Peak positions give information about interplanar distances. This information can be compared with known standards for identification of phases present in the sample.
Structure Determination Methods
Structure determination in X-ray diffraction spectroscopy involves methods that convert diffraction data into 3D structural information under the assumption of periodic arrangement of atoms in a crystal. Common methods include:
- Direct methods: These exploit the relationship between observed intensity and atomic structure. They are often used for small crystals.
- Reciprocal space methods: Techniques like Fourier transforms can be applied to convert the diffraction pattern into real-space atomic arrangements. It's powerful for elucidating complex structures.
- Patterson methods: This is useful particularly when some prior information about the structure is known. It aids in locating heavy atoms within the crystal structure.
Rietveld Refinement
Rietveld refinement is a sophisticated method for analyzing X-ray diffraction data from polycrystalline materials. It allows for simultaneous refinement of multiple parameters, resulting in a detailed characterization of the sample. Key features of this method include:
- Simultaneous fitting: The Rietveld method fits the entire diffraction pattern, rather than analyzing individual peaks. This means it can accommodate various phases and structural changes within the sample.
- Parameter optimization: Various parameters, like atomic positions, thermal vibrations, and background values, are refined iteratively, allowing for highly accurate structural models.
- Complexity handling: Rietveld refinement can handle samples with a high degree of complexity, making it valuable for modern materials' analysis.
In summary, data analysis in X-ray diffraction spectroscopy is indispensable for accurately interpreting the structure and properties of materials. By mastering the techniques of peak identification and fitting, structure determination methods, and Rietveld refinement, researchers can unlock a wealth of information about crystalline substances.
Applications of X-Ray Diffraction Spectroscopy
X-ray diffraction spectroscopy plays a pivotal role in various scientific fields. Its applications contribute significantly to advancements in materials science, chemistry, biology, and pharmaceuticals. Understanding these applications helps researchers appreciate the potential of this technique and its impact on modern science.
Materials Science
In materials science, X-ray diffraction is essential for analyzing the structure of materials. This technique enables scientists to determine crystallographic information, which is vital for understanding material properties. For instance, the investigation of phase transitions or the formation of new materials heavily relies on this analysis.
Moreover, X-ray diffraction aids in identifying defects in crystalline structures. Researchers can study how these defects affect mechanical properties such as strength, ductility, and hardness. This information is crucial in developing new materials with tailored properties, allowing for innovations in industries ranging from aerospace to electronics.
Chemistry and Catalysis
In chemistry, X-ray diffraction is valuable for characterizing complex molecular structures. This method is particularly beneficial in studying catalysts, which are substances that accelerate chemical reactions. By providing detailed structural data, researchers can gain insights into how catalysts work and their efficiency in facilitating reactions.
The ability to identify the arrangement of atoms in a molecule helps chemists design better catalysts, improving reaction rates and selectivity. This advancement is crucial for developing sustainable chemical processes, where efficiency and minimal waste are of utmost importance.
Biological Applications
X-ray diffraction also finds significant applications in biological sciences. This technique helps in determining the structures of biomolecules, such as proteins and nucleic acids. Understanding the three-dimensional arrangement of these molecules is essential for elucidating their functions in biological systems.
For example, the determination of protein structures supports drug design efforts. Knowing how a drug interacts with its target protein allows for the rational design of compounds that can effectively modulate biological activity. This information is vital in medical research and therapeutic development.
Pharmaceuticals
In pharmaceuticals, the applications of X-ray diffraction are numerous. This technique assists in the identification and characterization of pharmaceutical compounds. Accurate determination of crystalline structures helps ensure the efficacy and safety of drugs.
Additionally, X-ray diffraction is instrumental in polymorphism studies of drugs, which involves understanding different crystal forms. Each polymorph can exhibit distinct physical and chemical properties. Researchers leverage this knowledge to optimize drug formulations, enhancing solubility and bioavailability.
Recent Advances in X-Ray Diffraction Spectroscopy
Recent advancements in X-ray diffraction spectroscopy (XRD) have significantly changed how researchers analyze and interpret material structures. These innovations not only enhance the capabilities of XRD but also elevate its importance across several scientific fields. By integrating novel technologies and methodologies, researchers can now achieve better precision and efficiency in material characterization.
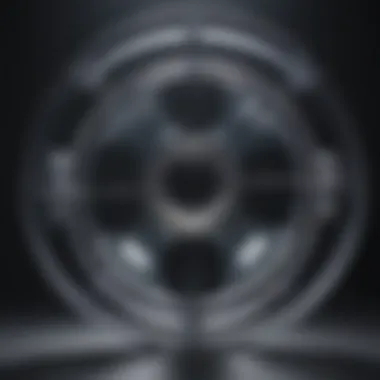
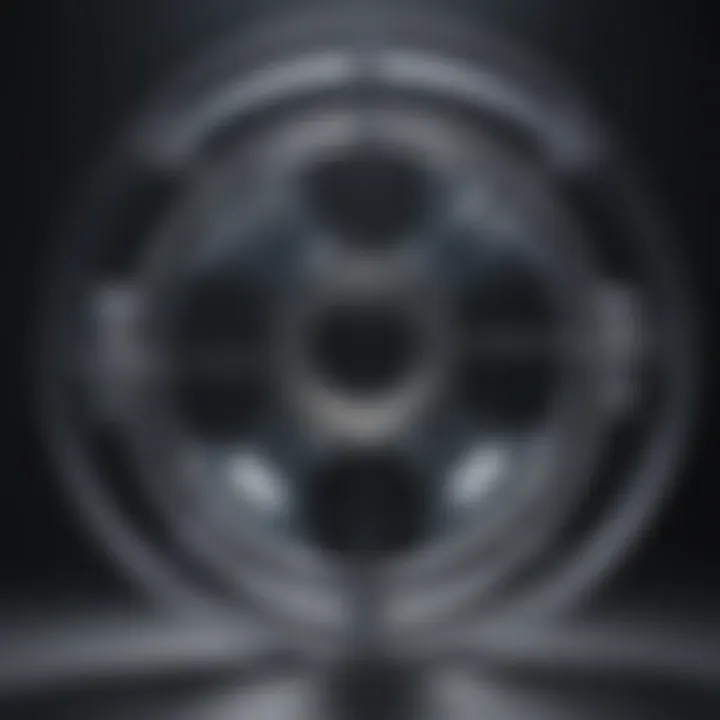
Technological Innovations
Technological innovations play a pivotal role in the evolution of X-Ray diffraction spectroscopy. Some key advancements include:
- High-Throughput XRD: This allows for rapid analysis of large sample sets. It improves productivity by decreasing the time needed for measurements.
- 2D Detectors: These detectors provide comprehensive data collection, reducing data collection time while improving resolution significantly. This leads to faster peak identification and more accurate structural analyses.
- Synchrotron Sources: The use of synchrotron radiation has enhanced the brilliance of X-ray sources, resulting in improved resolution and sensitivity. This enables scientists to investigate smaller samples or analyze at higher resolutions than conventional X-ray tubes.
- In-Situ XRD: In-situ techniques offer the ability to observe structural changes in real-time during material synthesis or modification. This is crucial for understanding dynamic processes that traditional methods cannot capture.
These innovations not only enhance the experimental capabilities but also broaden the range of applications for XRD in materials science, chemistry, and even biology.
Integration with Other Techniques
The integration of X-ray diffraction spectroscopy with other analytical techniques has opened new avenues for research. This is particularly important for comprehensive material characterization. Some notable integrated methods include:
- XRD and Scanning Electron Microscopy (SEM): Combining these techniques allows for both structural and morphological analysis at varying scales. XRD provides crystal structure insights while SEM reveals surface details and particle morphology.
- XRD coupled with Energy Dispersive X-ray Spectroscopy (EDX): This integration permits element-specific analysis alongside structural data. Understanding correlations between elemental composition and crystal structure is vital in various fields like materials science and catalysis.
- XRD with Atomic Force Microscopy (AFM): This combination enhances spatial resolution, allowing for the examination of surface topography at the nanoscale alongside the crystal structure analysis provided by XRD.
- XRD and Spectroscopy Techniques: Pairing XRD with techniques like Raman or IR spectroscopy offers comprehensive insights into vibrational modes and chemical bonding in materials, supplementing the structural information obtained through XRD.
By integrating XRD with other analytical methods, researchers can address complex questions about materials that single techniques often cannot answer effectively.
In summary, the recent advances in X-ray diffraction spectroscopy demonstrate the continuous evolution of this technique. The technological innovations and integrations with other methods enhance its relevance and application in various scientific areas. As research pushes the boundaries of material science, understanding these advances is crucial for students and professionals involved in XRD.
Limitations of X-Ray Diffraction Spectroscopy
Understanding the limitations of X-ray diffraction spectroscopy is crucial for researchers and practitioners in this field. These limitations impact not only the quality of the data obtained but also the interpretation of that data in various applications. A clear awareness of the challenges can lead to better experimental designs and more accurate analyses, ensuring that the findings can be relied upon in both scientific and industrial applications.
Sample Preparation Challenges
Sample preparation plays a significant role in X-ray diffraction spectroscopy. The quality of the sample directly influences the accuracy and reliability of the results. There are several challenges:
- Homogeneity: Achieving a homogeneous sample is essential for reliable diffraction patterns. Variations in composition can lead to inaccuracies in peak positions and intensities.
- Size and Shape: Samples need to be of suitable size and shape. For example, in powder X-ray diffraction, the particles should be fine enough to allow for proper X-ray penetration, yet not so fine that they agglomerate.
- Alignment: In single-crystal X-ray diffraction, precise alignment of the crystal is required. Misalignment can produce skewed data and complicate structure determination.
- Moisture Control: Many materials absorb moisture, altering their structure and diffraction properties. Proper drying methods are necessary to maintain sample integrity.
Researchers need to be aware of these challenges and may often have to optimize their preparation protocols to produce satisfactory results. Inadequate preparation can lead to misleading data, which can affect subsequent analysis and conclusions.
Resolution and Detection Limits
Resolution and detection limits are critical factors in determining the efficacy of X-ray diffraction spectroscopy. They dictate what can and cannot be detected within a sample, as well as the precision of the results:
- Crystal Size: The size of the crystals in the sample plays a vital role in resolution. Smaller crystals lead to broader peaks in the diffraction pattern, making it difficult to resolve complex structures.
- Instrumental Factors: The resolution can vary with the configuration of the X-ray source, the quality of the optics, and the specifications of the detector. Higher resolution often comes at the cost of longer measurement times and more complex data processing.
- Intensity Limitations: Weakly diffracting samples can produce low-intensity signals, which may fall below the detection threshold of the instrument, leading to missed information about the sample structure.
- Overlapping Peaks: In complex samples, the presence of overlapping diffraction peaks can complicate analysis, making it difficult to distinguish between different phases or components.
Awareness of these resolution and detection limitations is important for interpretting results correctly. Researchers should perform preliminary tests to determine if their samples are suitable for the desired analysis.
Future Perspectives on X-Ray Diffraction Spectroscopy
The future of X-ray diffraction spectroscopy (XRD) holds significant promise for expanding our understanding of the material world. As technology progresses, so too will the methods and applications of XRD. This section focuses on emerging trends in research and potential new applications that could reshape the landscape of scientific inquiry.
Emerging Trends in Research
Innovations in XRD techniques are evolving rapidly. Precision and speed are becoming paramount. Researchers are increasingly utilizing synchrotron radiation and free electron lasers. These sources provide more intense and focused X-ray beams, allowing for detailed studies of complex materials.
One noteworthy trend is the advent of advanced data analysis techniques. The integration of machine learning algorithms is transforming how researchers interpret diffraction patterns. Machine learning can enhance peak identification, refine structures, and streamline data processing. These developments improve accuracy and reduce time spent on manual analysis.
Another significant trend is in the realm of in situ and operando studies. XRD is being adapted to observe transformations in materials under real-world conditions. This real-time analysis is essential for fields like catalysis and battery technology, where understanding dynamic changes is crucial.
Potential New Applications
As XRD technology advances, the potential for new applications broadens significantly. One promising area is in nanotechnology. The ability to analyze nanoscale materials with precision opens the door to new discoveries in both their structural and functional properties.
Biomaterials is another emerging field. With the growing interest in personalized medicine, the characterization of biomaterials at a molecular level using XRD can provide critical insights. This application can enhance the development of biocompatible materials for implants and drug delivery systems.
Additionally, the role of XRD is increasing in the realm of energy storage and conversion. As materials such as perovskites and lithium-ion battery components are studied, XRD will contribute to developing more efficient and sustainable technologies.
"X-ray diffraction spectroscopy continues to push the boundaries of material analysis, fostering new insights and applications across a variety of scientific fields."
Overall, the future perspectives for X-ray diffraction spectroscopy reveal an evolving landscape. With continuous advancements and innovative applications, XRD is set to remain a critical tool in understanding the fundamental nature of materials.
Closure
The conclusion of this article encapsulates the significance of X-ray diffraction spectroscopy (XRD) in contemporary scientific pursuits. The exploration of this technique reveals its extensive applications, particularly in materials science, biology, and chemistry. The principles discussed throughout the article illuminate the underlying mechanisms that allow researchers to analyze the crystalline structure and material properties effectively.
Summary of Key Points
In reviewing the key points related to X-ray diffraction spectroscopy, several pivotal aspects come to light:
- Fundamental Principles: The interaction of X-rays with matter forms the basis of XRD. It is crucial to understand Bragg's Law and how X-rays are produced.
- Instrumentation: Key components such as X-ray sources, detectors, and goniometers play critical roles in obtaining accurate data.
- Data Analysis: Techniques for peak identification and Rietveld refinement are vital for interpreting the resulting diffraction patterns.
- Applications: Diverse fields utilize XRD, ensuring its relevance in chemical analysis, material characterization, and biological studies.
- Future Trends: Emerging technologies and potential applications indicate that XRD will continue to evolve and contribute to various scientific advancements.
The Importance of XRD in Modern Science
X-ray diffraction spectroscopy holds immense importance in todayβs scientific landscape. It not only provides insights into material properties but also aids in the development of new materials and systems. Here are several considerations:
- Material Characterization: Through crystal structure analysis, XRD helps in the discovery and optimization of materials, leading to advancements in electronics, catalysis, and pharmaceuticals.
- Quality Control: In industries such as pharmaceuticals, XRD serves as a critical tool for quality assurance, ensuring that products meet strict standards.
- Interdisciplinary Applications: The versatility of XRD allows for its integration across various fields, thereby accelerating interdisciplinary research.
- Technological Advancements: With ongoing innovations in detection and analysis techniques, XRD is poised to become even more valuable for future scientific inquiries.
"X-ray diffraction not only revolutionizes the analysis of crystal structures but also continuously pushes the boundaries of scientific exploration."