Exploring Orbital Science: Principles and Applications
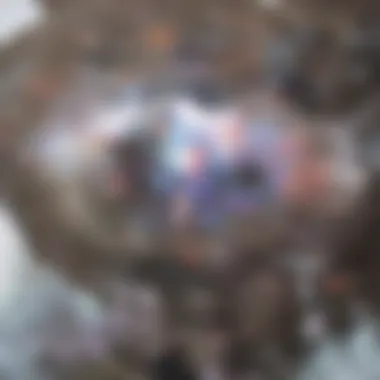
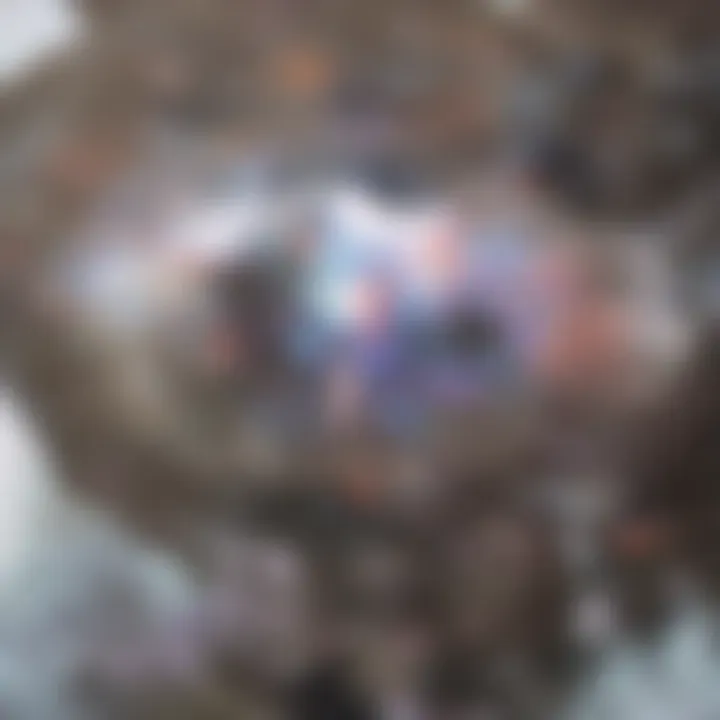
Intro
Orbital science plays a crucial role in our understanding of the cosmos, integrating principles of physics, engineering, and astronomy. As we explore the mechanics of celestial movements, we uncover valuable insights into the behavior of objects under gravitational influence. This field of study not only contributes to our comprehension of the universe but also enhances technological innovations. In this article, we will delve into the key principles, relevant technologies, and real-world applications of orbital science, revealing its significance and future challenges as it relates to satellite deployment, space missions, and the growing concern of space debris.
Research Background
Overview of the Scientific Problem Addressed
Understanding orbital dynamics is essential for navigating and utilizing space effectively. The fundamental challenge lies in predicting the trajectories of various celestial objects, including satellites, comets, and asteroids. This involves both theoretical models and practical applications that can adapt to the unique conditions of space. As technology advances, the need for precise calculations and assessments becomes increasingly vital for governments, scientists, and private enterprises involved in space exploration.
Historical Context and Previous Studies
The study of orbital mechanics began with the insights of Johannes Kepler, who formulated the laws of planetary motion in the early 17th century. His work laid the groundwork for Isaac Newton's universal law of gravitation. Since then, the field has evolved significantly, incorporating advances in mathematics, physics, and engineering. Notable studies include the development of classical mechanics, the introduction of numerical methods for solving complex orbital equations, and the emergence of computational models that simulate orbital behavior. Modern initiatives, including the Precision Orbit Determination by NASA, exemplify ongoing research aiming to refine our understanding of orbital mechanics.
Findings and Discussion
Key Results of the Research
Research in orbital science has led to various advancements in satellite technology, propulsion systems, and tracking methods. Key findings indicate that the complex interactions among gravitational forces and environmental factors, such as atmospheric drag and radiation pressure, significantly influence the trajectories of orbital bodies. Moreover, data from missions like the European Space Agency's Gaia project contribute to a more accurate mapping of celestial objects and their paths.
Interpretation of the Findings
These findings underscore the importance of integrating continuous data collection methods with sophisticated modeling techniques to enhance precision in orbital predictions. The implications extend to various applications, including mission planning, collision avoidance for satellites, and long-term predictions for space debris management. As researchers continue to tackle these challenges, the collaboration across disciplines will become imperative to address both current needs and future explorations in space.
"Understanding stellar movements is not just a scientific endeavor; it embodies our quest to unlock the mysteries of the universe."
Prolusion to Orbital Science
Orbital science plays a critical role in comprehending not only the movement of celestial bodies but also the technology that enables exploration of these entities. Understanding these dynamics allows for advancements in satellite technology, space travel, and even our comprehension of planetary systems. Exploration of orbital science can lead to more efficient satellite deployments and improved communication systems. This section serves as a foundation for understanding how these principles interlink with the vast universe around us.
Historical Context
The journey into orbital science began long before the advent of modern spacecraft. Early astronomers, such as Johannes Kepler and Isaac Newton, laid the groundwork with their laws of planetary motion and gravitation. Kepler, in the early 17th century, described how planets move in ellipses rather than perfect circles. This was a shift in understanding that spurred further inquiry.
In the 20th century, with the launch of Sputnik by the Soviet Union in 1957, the field gained momentum. This satellite’s successful orbit opened avenues for exploration and understanding that many had only dreamt of. The Apollo missions in the late 1960s further solidified human presence in space, showcasing the practical applications of orbital science. These pivotal moments in history not only changed our scientific approach but also ignited public interest in space exploration.
Importance of Orbital Science
Orbital science is significant for various reasons. It enhances our ability to track and understand satellite movements, which is essential for modern communication networks and GPS systems. The technology developed through orbital science helps address practical issues like climate monitoring.
Moreover, orbital science is essential for future explorations. By studying orbital mechanics, scientists can design missions that minimize fuel use and reduce travel time to distant celestial bodies.
Through the understanding of orbits, we can also appreciate the impact of space debris and devise methods for its mitigation. This includes advancements in policy and international cooperation to ensure sustainable exploration. In summary, the insights gained from orbital science serve as a foundation for technological and scientific growth, making it a crucial field of study for students, researchers, and professionals alike.
The essence of orbital science extends beyond just celestial mechanics; it encompasses the global challenges we face in our quest for sustainability in space.
Fundamental Principles of Orbital Mechanics
The study of orbital mechanics is fundamental to our understanding of celestial movements. It lays the groundwork for various applications, from satellite deployment to space exploration. Orbital mechanics guides engineers and scientists as they design missions and understand how different forces act on objects in space. Without these principles, the ability to send and maintain objects in orbit would be severely limited.
Kepler's Laws of Planetary Motion
Johannes Kepler formulated three laws in the early 17th century that describe the motion of planets around the sun. These laws provide crucial insights into orbital paths:
- The Law of Ellipses: Planets move in elliptical orbits with the sun at one focus. This law highlights the non-circular nature of orbits, which is essential for accurate modeling.
- The Law of Equal Areas: A line segment joining a planet and the sun sweeps out equal areas during equal intervals of time. This implies that the speed of a planet changes depending on its distance from the sun.
- The Law of Harmonies: The square of a planet's orbital period is proportional to the cube of the semi-major axis of its orbit. This law establishes a relationship between the distance of planets and their motion, leading to methods for calculating orbital characteristics.
These laws are not only pivotal in astronomy but also serve as a foundation for modern satellite dynamics. They assist satellite engineers in determining how to maintain the desired trajectories for various missions.
Newton's Law of Universal Gravitation
Sir Isaac Newton's contribution to orbital mechanics cannot be understated. His law of universal gravitation states that every point mass in the universe attracts every other point mass with a force that is proportional to the product of their masses and inversely proportional to the square of the distance between them. This gravitational force dictates the motion of celestial bodies and explains why orbits happen.
The implications of this law are profound:
- It allows scientists to understand how planets, moons, and other bodies interact with one another.
- It forms the basis for calculating trajectories in orbital missions, ensuring smaller objects can maintain stable orbits around larger ones, such as Earth or Mars.
Orbital Energy and Stability
Understanding the concepts of orbital energy and stability is critical in orbital mechanics. An object's total mechanical energy is the sum of its kinetic and potential energy. Kinetic energy relates to motion, while potential energy relates to its position in a gravitational field. These energy forms influence an orbit's shape and stability.
An orbit can be classified into several types based on its energy considerations:
- Bound Orbits: These orbits have negative total energy, such as circular and elliptical orbits. They are stable and will continue unless acted upon by an external force.
- Unbound Orbits: These orbits have positive total energy, including parabolic and hyperbolic trajectories. An object on such paths will escape the gravitational influence of a planet.
Stability is also linked to perturbations caused by external forces, such as gravitational influences from other celestial bodies or atmospheric drag in low Earth orbits. Orbital mechanics must take these factors into account to ensure long-term mission success.
"The principles of orbital mechanics not only inform our understanding of celestial dynamics but also underscore the technological feats of satellite launches and deep-space missions."
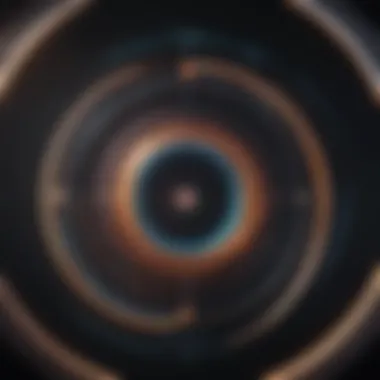
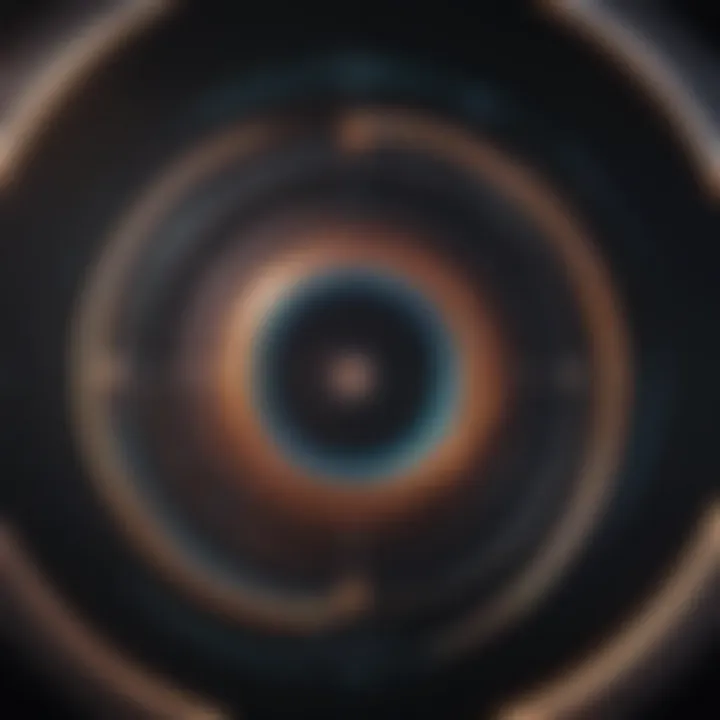
Types of Orbits
Understanding the various types of orbits is critical in the field of orbital science. Each type of orbit serves distinct purposes, influenced by elements like altitude, inclination, and application. From deploying satellites to conducting scientific research, the choice of orbit impacts performance and mission success. Knowing which orbit to use ensures optimal functionality of space missions.
Geostationary Orbit
The geostationary orbit is a unique position where satellites remain fixed above a specific point on Earth. This orbit is approximately 35,786 kilometers above the equator. The satellite matches the Earth’s rotation, allowing it to maintain a constant position relative to the surface. This characteristic is crucial for communication satellites, as it permits continuous coverage over a region without interruption.
One major advantage of this orbit is that it provides a stable platform for data transmission and weather observation. However, it is limited to the equatorial region, which means its usefulness diminishes in areas far north or south.
Low Earth Orbit
Low Earth Orbit (LEO) is situated between 160 to 2,000 kilometers above the Earth. Many important missions occur here. LEO is popular for several reasons. Its proximity allows for lower launch costs and shorter communication delays. Most manned space flights and recent satellite constellations, like Starlink, utilize this orbit.
Operational benefits are numerous. Satellites in this region can capture high-resolution images and gather data efficiently due to their closeness to Earth. However, satellites in LEO face challenges regarding atmospheric drag and orbital decay, necessitating regular adjustments.
Polar Orbit
The polar orbit passes over the Earth's poles, allowing satellites to scan the entire surface over multiple orbits. This path is particularly useful for Earth observation satellites, as it captures comprehensive data on weather patterns, environmental changes, and land use.
Satellites in a polar orbit can provide frequent updates as they pass over the changing surface of the Earth. A disadvantage is the longer time taken for revisit intervals. Despite this, the ability to view every part of the globe makes polar orbits indispensable for various scientific missions.
Elliptical Orbits
Elliptical orbits are characterized by their oval shape, varying distance from the Earth during different points in the orbit. This type of orbit can be beneficial for several applications, particularly in remote sensing and scientific experimentation. One well-known example of elliptical orbit is the highly elliptical orbits used by satellites like the Molniya, which is designed to spend most of its time over high latitudes.
These orbits allow satellites to remain over areas of interest for more extended periods, helping with data collection when positioned optimally. However, entering and exiting such orbits requires meticulous planning to manage changes in velocity and altitude correctly.
Tools and Technologies in Orbital Science
The domain of orbital science is deeply intertwined with a myriad of tools and technologies. These advancements are crucial for the study, exploration, and utilization of orbits. They serve various purposes—from satellite communication to astronomical observations. Understanding these technologies can shed light on how we have reached the current stage of exploration of our cosmic environment.
Satellite Technology
Satellite technology stands as a cornerstone in orbital science. Satellites are designed to orbit Earth or other celestial bodies for numerous applications. They are vital for communication, weather forecasting, navigation, and Earth observation. For instance, communication satellites facilitate global telecommunication networks by transmitting signals across vast distances.
Satellites are classified into various categories based on their functions:
- Geostationary Satellites maintain a position relative to the Earth, which simplifies communication.
- Low Earth Orbit Satellites are instrumental in capturing high-resolution images of the Earth’s surface.
- Scientific Satellites like the Hubble Space Telescope operate beyond Earth's atmosphere, providing invaluable data on celestial phenomena.
In addition to their diverse functionalities, advancements in satellite design and technology continue to push the boundaries of what is achievable. Smaller, more cost-effective satellite designs, known as CubeSats, are becoming popular. Their accessibility allows more institutions and organizations, including universities, to participate in space research.
Space Probes and Rovers
Space probes and rovers are essential for exploring environments that are too distant or inhospitable for human presence. Probes such as Voyager and New Horizons have journeyed far beyond the solar system, gathering data about planets and their moons. This information plays a key role in expanding our knowledge about the cosmos.
Rovers, on the other hand, are deployed on the surfaces of planets and moons. Notable examples include the Mars Curiosity rover and NASA's Perseverance rover. These machines analyze soil and rock samples, assess environmental conditions, and search for signs of past life. The results from these missions have profound implications for our understanding of both planetary science and the potential for extraterrestrial life.
Tracking and Measurement Systems
Accurate tracking and measurement systems are vital for ensuring the success of missions in orbital science. These systems can monitor the location and trajectory of satellites and spacecraft in real time. GPS technology, for example, provides precise positioning, which is crucial for navigation and data collection.
Additionally, radar and ground-based tracking systems assist in gathering data on objects in orbit. They help optimize flight paths and avoid collisions with space debris, which is an increasing concern in our increasingly cluttered orbital environment.
Investments in advanced tracking technology enhance our capabilities to manage and mitigate risks associated with space exploration. Effective measurement systems will likely continue to evolve, incorporating artificial intelligence to process vast datasets efficiently.
"Each of these tools and technologies plays a pivotal role in advancing our understanding of space, enabling coordinated actions and increasing safety."
In summary, tools and technologies in orbital science are not merely aids; they are fundamental components of the exploration framework. They shape how we communicate, navigate, and uncover the mysteries of our universe.
Satellite Deployment and Operations
Satellite deployment and operations are critical components in the realm of orbital science. This field involves the know-how to launch, position, and manage satellites to ensure they fulfill their intended functions. Understanding these processes is essential for optimizing satellite performance and enhancing the capabilities of various applications. From communication to Earth observation, the importance of deploying satellites correctly cannot be overstated.
Launch Vehicles
Launch vehicles, commonly referred to as rockets, play an essential role in transporting satellites from the Earth's surface into orbit. The design and capabilities of these vehicles vary greatly, often tailored to specific types of missions and payloads. Notably, rockets like SpaceX's Falcon 9 and United Launch Alliance's Atlas V have revolutionized satellite deployment by reducing costs and increasing reliability.
- Types of Launch Vehicles
- Factors in Selecting Launch Vehicles: When choosing a launch vehicle, mission planners must consider payload size, destination orbit, and cost. The right choice can enhance mission success rates and overall efficiency.
- Expendable Launch Vehicles (ELVs): These are single-use rockets that discard stages after each use. Examples include the Ariane 5 and Delta IV Heavy.
- Reusable Launch Vehicles (RLVs): These rockets can return to Earth for refurbishment and subsequent flights. SpaceX's Falcon 9 is a prime example that has significantly impacted the accessibility of space.
Orbital Insertion Strategies
Once a satellite is launched, the next step involves establishing its orbit. Orbital insertion is a critical phase that requires precision and expertise. Two primary strategies exist for orbital insertion: direct insertion and multi-burn insertion.
- Direct Insertion: In this method, the satellite is placed into its final orbit in one continuous burn. This is common for smaller payloads that can achieve their target efficiently.
- Multi-Burn Insertion: Larger satellites, such as those intended for geostationary orbit, typically employ this strategy. In this case, several propulsion maneuvers are conducted to gradually shift the satellite into its desired position.
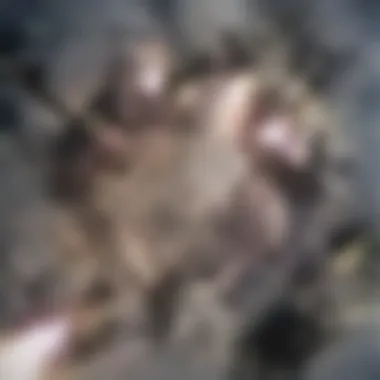
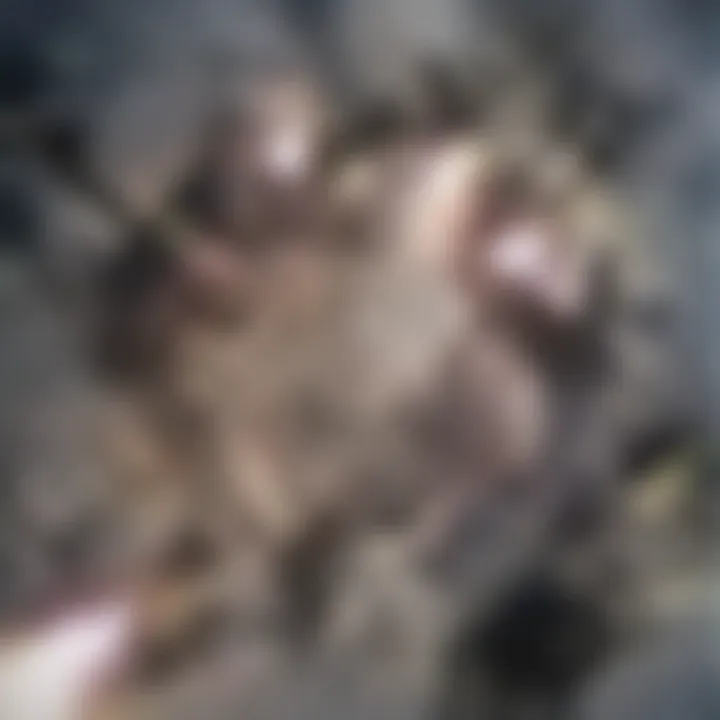
An accurate insertion is necessary to avoid costly adjustments later. Failure to do so can lead to increased operational expenses and reduced satellite lifespan.
End-of-Life Procedures
End-of-life procedures are a vital aspect of satellite operations that are often overlooked. Once a satellite has completed its mission, it must be responsibly decommissioned to minimize the creation of space debris. International guidelines, such as the United Nations Committee on the Peaceful Uses of Outer Space frameworks, lay out best practices for these processes.
- Deorbiting: This method involves bringing the satellite down into the Earth's atmosphere to burn up upon re-entry. It is recommended for satellites in low Earth orbit.
- Disposal Orbits: Satellites in higher orbits, particularly geostationary satellites, may move to a designated "graveyard" orbit. This ensures that active orbits remain clear for new satellites.
End-of-life procedures contribute to sustainable space exploration and help maintain the safety of orbital environments.
"As the number of satellites increases, responsible end-of-life strategies become essential for mitigating space debris risks."
Space Missions and Their Impact
Space missions represent a crucial element of orbital science, embodying the practical application of theoretical principles to explore and understand our universe. They provide opportunities not only for scientific discovery but also for technological advancement and international collaboration. The significance of space missions lies in their dual capacity to enhance our comprehension of celestial phenomena and to drive innovations that benefit life on Earth.
Space missions yield numerous benefits, including:
- Scientific Knowledge: Missions help gather data about planets, stars, and other celestial bodies, contributing to the understanding of fundamental astrophysical processes.
- Technological Innovation: The challenges of space exploration often lead to the development of new technologies. Many advancements, such as satellite technology and materials science, have found applications on Earth.
- International Collaboration: Space missions often involve cooperation between nations, fostering relationships and shared goals within the global community.
However, ethical considerations and the sustainability of space activities also play a vital role in shaping policies and methodologies regarding missions.
Historical Missions Overview
The journey of space exploration began with significant milestones that laid the foundation for current and future endeavors. The launch of Sputnik 1 by the Soviet Union in 1957 marked the start of the space age. It was the first artificial satellite, circling the Earth and offering a glimpse into the potential of orbital science.
Following this, the Apollo Program symbolized a monumental achievement in human spaceflight. The lunar landings, especially Apollo 11 in 1969, showcased the ability to send humans beyond Earth and return safely. This mission not only ignited public interest but also inspired generations of scientists and engineers.
Other missions, such as Voyager 1 and Voyager 2, expanded our understanding of the outer planets. They traveled beyond the solar system, sending back invaluable data about planetary atmospheres and magnetic fields.
Current Missions and Objectives
As of today, the landscape of space missions continues to evolve, with multiple agencies and private companies embarking on innovative projects. One noteworthy effort is NASA's Perseverance rover, which landed on Mars in 2021. Its main objectives include searching for ancient life forms and collecting rock samples for future return to Earth. This is a critical step towards human exploration of Mars and has potential implications for understanding potential life on other planets.
In addition, the James Webb Space Telescope, launched in late 2021, aims to provide deeper insights into the universe. With its advanced technology, it will help scientists examine the formation of stars, galaxies, and the atmospheres of exoplanets.
Future Mission Prospects
Looking ahead, the future of space missions promises exciting developments. Plans for missions to establish human presence on Mars are already in the works, with efforts from SpaceX and NASA contingent on advancements in sustainable life support and habitat technologies. Such missions are not merely exploratory but could pave the way for colonization.
Additionally, concepts like asteroid mining are garnering attention, with the potential for extracting valuable resources. The utilization of robotic probes for these endeavors may revolutionize resource management in space.
Lastly, international collaborations may expand further. Projects like the Lunar Gateway, a planned space station in lunar orbit, promise to unite nations for sustainable exploration beyond Earth.
"The impact of space missions extends beyond mere exploration; they are a harbinger of future innovations and international cooperation."
Challenges in Orbital Science
The field of orbital science faces numerous challenges that are critical for its continued advancement and relevance. Addressing these challenges not only enhances our understanding of orbital mechanics but also ensures the safety and sustainability of space operations. Among the most pressing issues are space debris mitigation, the need for international cooperation in policy formulation, and the limitations posed by current technologies. Each of these elements holds significant implications for the future of space exploration and satellite operations.
Space Debris Mitigation
Space debris is a growing concern in orbital science, with thousands of defunct satellites and fragments from past missions cluttering Earth's orbit. This debris poses a risk to active satellites and manned missions, potentially leading to catastrophic collisions. The significance of space debris mitigation lies in developing strategies to manage and reduce this hazard.
- Collision Avoidance: Implementing tracking systems and predictive models can help in anticipating potential collisions and adjusting satellite trajectories accordingly.
- Debris Removal Technologies: Various concepts, like capturing debris using robotic arms or nets, are being explored. This technology needs extensive testing to ensure efectiveness and viability.
- End-of-Life Protocols: Ensuring satellites are deorbited after their operational life can minimize long-term debris generation.
International Cooperation and Policy
The challenge of orbital science transcends national boundaries, making international cooperation indispensable. Space is a shared domain, and understanding orbital mechanics and safety protocols require collective efforts. Key aspects of this cooperation include:
- International Treaties: Existing treaties, such as the Outer Space Treaty, provide a legal framework, but they may require updates to address modern challenges.
- Joint Missions: Collaborative missions, such as those between NASA and ESA, can pool resources and expertise to tackle complex problems more efficiently.
- Standardization of Practices: Developing global standards for satellite design, operation, and end-of-life procedures can enhance safety and sustainability in space.
Technological Limitations
Technological advancements are vital in overcoming many challenges in orbital science, yet limitations still persist. The field needs continuous investment in research and innovation. Some notable areas of concern are:
- Propulsion Technology: Current propulsion systems often restrict orbit maneuvers and debris avoidance. More efficient alternatives, such as electric propulsion, show promise but need further development.
- Data Processing and Analysis: The vast amounts of data gathered from satellite monitoring present challenges in real-time processing and analysis. Robust systems must be established to utilize this data effectivley.
- Satellite Lifespan and Durability: Developing satellites that can withstand the harsh conditions in space for extended periods is crucial. Advances in materials science and engineering practices are necessary to ensure longevity and reliability.
Overall, the challenges in orbital science encompass a wide range of considerations that must be addressed to ensure the orderly exploration and utilization of space. A proactive approach to these issues will not only safeguard space operations but also pave the way for future innovations in the field.
Theoretical Perspectives in Orbital Science
Understanding the theoretical perspectives in orbital science aids in unearthing complex celestial dynamics and allows for accurate predictions of orbital behaviors. This section delves into the mathematical constructs and predictive methodologies that underpin our grasp of orbital mechanics. The emphasis on these theoretical elements demonstrates their critical role in enhancing our comprehension of space phenomena and their applications in real-world scenarios.
Mathematical Modeling of Orbits
Mathematical modeling plays a foundational role in orbital science. It provides a framework for representing the behavior of celestial bodies under the influence of gravitational forces. The essence of these models lies in their ability to encapsulate the physical laws governing motion.
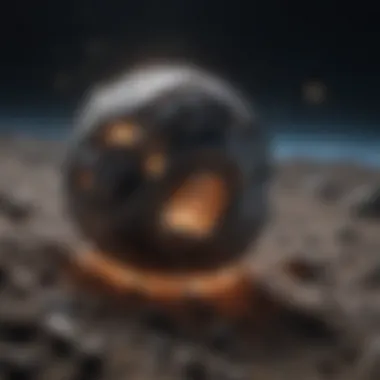
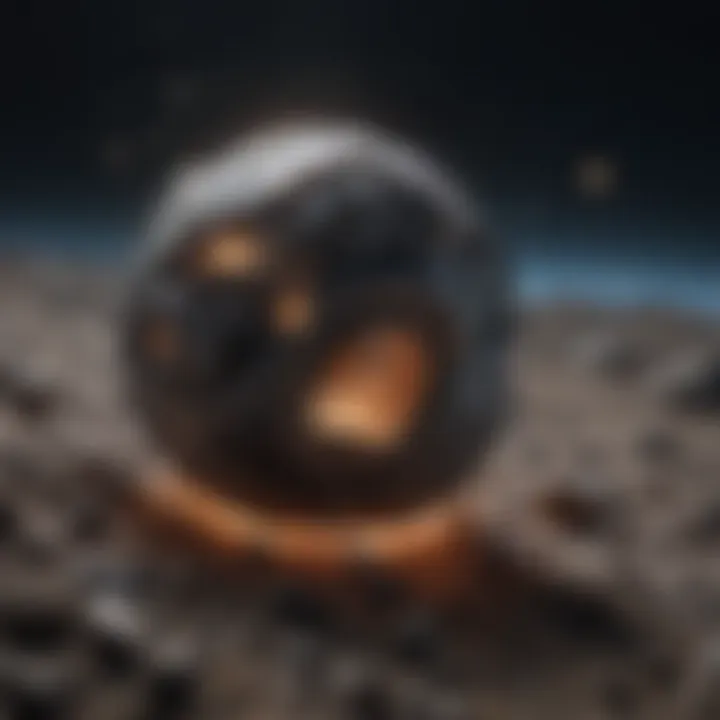
Some key aspects include:
- Kepler's Laws: Formulated in the 17th century, these laws describe how planets orbit around the sun. They serve as a basis for predicting orbital paths.
- Newton's Equations of Motion: These equations reveal the dynamics governing the motion of objects. They are integral for calculating trajectories and the forces acting upon satellites or space probes.
- Numerical Simulations: Often, analytical solutions to orbital problems are impractical due to complexities. Numerically solving differential equations helps scientists simulate orbital movements.
Mathematical models not only assist in predicting orbits but also in designing missions. Accurate modeling ensures that spacecraft can reach their intended destinations efficiently.
Simulations and Predictive Analytics
Simulations and predictive analytics complement mathematical models in assessing orbital dynamics. They enable scientists to visualize and analyze complex systems. By employing computational tools, researchers can simulate interactions between multiple bodies in space—a key factor in mission planning.
Some important features of this aspect include:
- Dynamic Modeling: Real-time simulations consider factors such as gravitational perturbations and non-spherical mass distributions, which alter orbits.
- Long-Term Predictive Analysis: Tools such as the integrated orbit prediction techniques allow projections of orbital paths over extended periods. This is crucial for contemplating satellite longevity and end-of-life scenarios.
- Interdisciplinary Collaboration: The integration of data from physics, engineering, and computer science enhances the accuracy of simulations. This cross-disciplinary approach yields a deeper understanding of orbital phenomena.
"The application of simulations in orbital science is indispensable for preparing for contingencies and understanding long-term orbital behaviors."
In sum, both mathematical modeling and simulations form the backbone of theoretical perspectives in orbital science. They not only augment our scientific knowledge but also pave the way for practical applications in satellite deployment and space exploration.
Case Studies in Orbital Science
Case studies in orbital science serve as a vital bridge between theoretical principles and practical applications. They provide tangible examples of how concepts discussed previously come to life through real-world missions. These case studies not only highlight significant advancements in technology and research but also offer insight into the challenges faced in space exploration. By analyzing specific missions, we gain a better understanding of both successes and setbacks in the field of orbital science. This understanding is crucial for future initiatives and for refining existing technologies. This article discusses two pivotal case studies: the Hubble Space Telescope and Mars Rover missions.
Hubble Space Telescope
The Hubble Space Telescope stands as a monumental achievement in both engineering and scientific inquiry. Launched in 1990, Hubble operates in low Earth orbit, circumventing atmospheric interference to deliver clear images of deep space. Its design integrates advanced optics with sensitive sensors, making it possible to observe cosmic phenomena ranging from exoplanets to distant galaxies.
Hubble's contributions to astronomy are profound. It has played a key role in measuring the expansion rate of the universe, providing evidence for dark energy, and enhancing our understanding of black holes. As a case study, Hubble exemplifies the interplay between technology and scientific discovery. Its ongoing upgrades and servicing missions underscore the importance of adaptability in space technologies. NASA has conducted several servicing missions to replace instruments and extend its operational lifespan.
"The Hubble Space Telescope has fundamentally altered our understanding of the universe."
In addition, Hubble highlights important considerations regarding collaboration in science. It is not only a product of NASA but also a partnership with the European Space Agency. This collaboration demonstrates the vital role of international cooperation in driving space exploration forward. Hubble has captured billions of images, making its data publicly available for research, education, and outreach. This accessibility fosters a global understanding of space and promotes further scientific inquiry.
Mars Rover Missions
Mars Rover missions represent a significant milestone in our exploration of other planets. These missions have provided invaluable data about the Martian environment and its history, enhancing our understanding of potential life beyond Earth. The most notable rovers, Spirit, Opportunity, Curiosity, and Perseverance, have each contributed uniquely to our knowledge of Mars.
Curiosity, for instance, has been equipped with advanced scientific tools to analyze soil samples and atmospheric conditions. Its discoveries, such as evidence of ancient water flows, have profound implications for understanding Mars' potential to support life. The current Perseverance rover adds another layer by collecting rock samples for future return missions to Earth.
These rover missions also illuminate the technical challenges faced in robotics and remote operation in harsh environments. The distance from Earth causes communication delays, demanding autonomous navigation and decision-making capabilities onboard.
Furthermore, Mars Rover missions exemplify long-term planning and international collaboration. Instruments from various countries contribute to the overall success of these missions. As we look toward future exploration, these case studies illustrate how past experiences can inform better designs and strategies.
In summary, studying specific missions like the Hubble Space Telescope and Mars Rovers provides essential insights into the principles and technologies of orbital science. They demonstrate the potential for human ingenuity in overcoming challenges and advancing our understanding of the universe.
Ethical Considerations in Orbital Science
As we advance our understanding of orbital science, ethical considerations become increasingly critical. The complexities of space exploration and technology bring significant responsibilities. The implications of our actions in space affect not only current generations but also future ones. Thus, a deliberate exploration of the ethical dimensions of orbital science is vital.
Balancing Scientific Progress with Ethical Concerns
Scientific progress in orbital science often pushes the boundaries of exploration and technology. However, rapid advancements raise ethical questions. For instance, the deployment of satellites impacts privacy and security. Data collected through satellites can reveal sensitive information about individuals and nations.
Moreover, as humanity ventures further into space, we must consider the potential disruption of celestial environments. There is a fear that mining asteroids or other bodies could lead to irreversible changes. Finding a balance between achieving scientific goals and ensuring ethical responsibility is crucial. Scientists, policy-makers, and ethicists must work together to devise frameworks guiding these advancements.
Access and Equity in Space Exploration
Space exploration should be a shared endeavor. However, access to the benefits of orbital science is not equal. Developed countries often dominate space missions and technologies, leaving developing nations behind. This disparity raises ethical concerns surrounding equity in space exploration.
Efforts to promote inclusivity should be prioritized. For example, international collaborations can help share the benefits of space technologies. Educational programs can empower students from less privileged backgrounds to participate in orbital science.
Addressing these concerns is essential for a just approach to intrinsic advancements. By ensuring broad access, we can promote a more equitable future in the realm of space exploration.
"Ethical considerations are not just an afterthought in science; they are integral to sustainable progress."
Closure
In examining orbital science, it is critical to consider its wide-reaching implications and the technologies that branch from it. The conclusion provides an opportunity to underline the overarching themes present throughout the article. As we reflect on the intricate relationship between celestial mechanics and technological innovation, we can appreciate how fundamental principles inform current practices and shape future advancements.
Recapitulation of Key Points
- Fundamental Principles: Our exploration began with the laws that govern orbital mechanics, such as Kepler's laws and Newton's gravitational principles. These form the backbone of orbital science.
- Types of Orbits: The article discussed various orbit classifications, including geostationary and low Earth orbits, which play vital roles in satellite deployment and global communication.
- Technologies and Tools: We examined how satellite technology, space probes, and tracking systems have evolved and transformed our understanding of space.
- Space Missions: Historical and current missions such as the Hubble Space Telescope and Mars rover initiatives showcased the applied nature of orbital science and its impact on knowledge acquisition.
- Challenges: Issues like space debris and international cooperation were highlighted, demonstrating the need for strategic approaches in managing orbital environments.
- Ethics and Considerations: Ethical implications have surfaced as essential discussions in balancing scientific exploration with moral responsibilities.
Vision for the Future of Orbital Science
Looking ahead, the future of orbital science promises to be dynamic and multifaceted. The combinations of emerging technologies and theoretical advancements prepare the ground for numerous possibilities:
- Sustainable Practices: As the concerns surrounding space debris grow, innovations aimed at debris removal and prevention will likely emerge as priority areas for development.
- International Collaboration: Future achievements may depend on the ability of nations to cooperate and establish common goals regarding space exploration and utilization.
- Interplanetary Missions: The goal of sending humans to Mars and other celestial bodies remains in focus, pushing the boundaries of our current capabilities.
- Advanced Technologies: Innovations in propulsion systems and AI-driven analytics will further enhance our ability to navigate and utilize orbital environments.
As these elements unfold, they provide not only a lens into the scientific community's aspirations but also a roadmap for students and professionals in the field.
"The exploration of space will carry on, driven by curiosity and the urgent need to expand our domain beyond Earth."
Through these discussions, we highlight the necessity of continuous learning and adaptability within orbital science, thereby enabling future explorers to tackle challenges with informed solutions.