Understanding mRNA and Its Role in Protein Synthesis
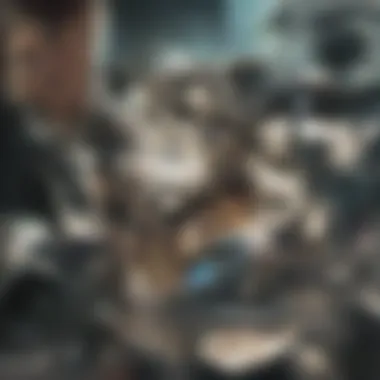
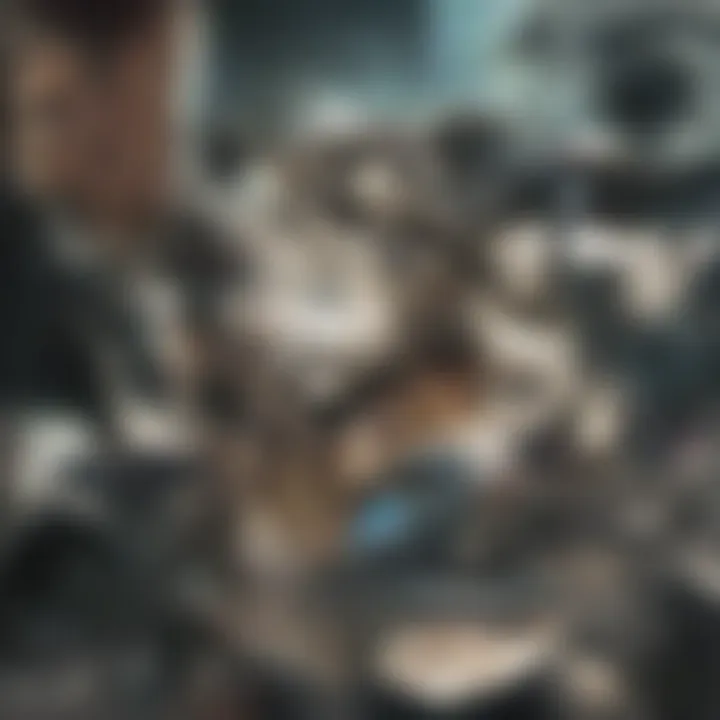
Intro
The study of mRNA and its role in protein synthesis holds significant relevance in modern biochemistry and genetics. This understanding lays the foundation for key advancements in biotechnology and medicine. In particular, mRNA sequences guide the assembly of amino acids, which ultimately form proteins that are essential for cellular structure and function.
Amino acids are the building blocks of proteins, and the sequence of these amino acids is determined by the mRNA transcribed from DNA. This intricate relationship between mRNA and proteins is a cornerstone of molecular biology. By exploring how mRNA sequences dictate amino acid assembly, we can better appreciate the complexities of gene expression and the subsequent biological processes.
Research Background
Overview of the Scientific Problem Addressed
The central issue in the study of mRNA is understanding how nucleotide sequences translate into functional proteins. The genetic code, consisting of codons—groups of three nucleotides—dictates this translation process. Each codon corresponds to a specific amino acid. Therefore, a comprehensive grasp of mRNA dynamics is critical for numerous applications, including therapeutic interventions and vaccine development.
Historical Context and Previous Studies
Historically, the elucidation of the role of mRNA began with key experiments in the mid-20th century. Researchers like François Jacob and Jacques Monod proposed the Messenger RNA hypothesis, which highlighted mRNA’s role in protein synthesis. Since then, studies have expanded, exploring various aspects of translation and transcription. More recent research focuses on the implications of mRNA technology in developing vaccines, such as those against COVID-19, demonstrating the practical significance of this field.
The direction of mRNA research has evolved, from understanding basic molecular mechanisms to embracing its implications in cutting-edge techniques.
"Understanding mRNA sequences is not just an academic exercise; it has real-world ramifications for medicine and biotechnology."
In summary, the discourse on mRNA and its implications is critical for various scientific fields and must be acknowledged as we advance our knowledge in genetics and molecular biology.
Prelude to mRNA
The exploration of mRNA is critical for understanding the fundamental processes of life. mRNA, or messenger RNA, serves as a pivotal intermediary in the process of gene expression. It takes the genetic information encoded in DNA and conveys it to the ribosomes, where proteins are synthesized. This mechanism is essential in cellular functions, making mRNA not just a participant in the process but a central player in the flow of genetic information.
Understanding mRNA encompasses various elements that facilitate protein synthesis. These elements include its structure, the transcription process, and its translation into functional proteins. Additionally, the implications of mRNA extend beyond the basic biological processes to practical applications in medicine and biotechnology. By comprehending mRNA, we unlock insights into genetic coding, protein formation, and potential therapeutic uses.
As we delve into the significance of mRNA, we will examine its definition and functions, along with a historical perspective on its discovery and research advancements. This foundation allows us to appreciate the complexity and importance of mRNA in contemporary scientific discussions.
Definition and Function of mRNA
Messenger RNA (mRNA) is a single-stranded nucleic acid molecule that conveys genetic information from DNA to the ribosome. The primary function of mRNA is to serve as a template for protein synthesis during the process of translation. This process involves specific sequences of nucleotides, which are interpreted by ribosomes to assemble amino acids in the correct order to form a protein.
The significance of mRNA lies not only in its role as a messenger but also in its ability to regulate gene expression. It can influence which proteins are produced and in what quantities. This regulation is crucial because proteins are responsible for a vast array of functions within living organisms, from catalyzing biochemical reactions to providing structural support.
Historical Context of mRNA Research
The concept of mRNA emerged in the early 1960s, marking a significant advancement in molecular biology. Instances of genetic material being transcribed into RNA were being researched widely, leading to the eventual identification of mRNA as a distinct molecule. The groundbreaking work of scientists like François Jacob and Jacques Monod in their exploration of gene regulation provided early insights into the function of mRNA.
Further research in the following decades elucidated the complexities of mRNA processes, including transcription and translation mechanics. Studies from the 1970s established linkages between mRNA sequences and amino acid coding, reinforcing the idea of a genetic code.
These historical developments laid the groundwork for the current understanding of mRNA, making it an area of intense research focus, particularly in the context of biotechnology and medicine today.
Transcription Process
Transcription is a fundamental biological process that involves the synthesis of RNA from a DNA template. This transition from DNA to RNA is crucial because it lays the groundwork for subsequent protein synthesis. Understanding transcription is essential to grasp the broader implications of how mRNA influences amino acid sequences, ultimately affecting cellular function and overall organismal biology.
The transcription process can be viewed as the first step in gene expression. It serves several important functions. First, it enables the creation of messenger RNA (mRNA), which carries genetic information from DNA to the ribosomes, where proteins are synthesized. Without transcription, cells would not be able to produce the proteins necessary for survival.
Mechanism of Transcription
The mechanism of transcription involves several key phases: initiation, elongation, and termination. This process begins when RNA polymerase binds to the promoter region of a gene on the DNA strand. The DNA unwinds, allowing the RNA polymerase to read the nucleotide sequence and synthesize a complementary RNA strand. During elongation, the RNA grows in length as nucleotides are added one after another. This phase continues until RNA polymerase encounters a termination signal, which prompts the release of the newly formed mRNA strand.
It is important to note that transcription is not simply a one-way street. The RNA molecule may undergo various forms of regulation, which include the action of transcription factors and other regulatory proteins that modulate the efficiency of this process. These factors can determine how much of a specific mRNA is produced, thereby influencing how much protein is eventually synthesized from that mRNA.
Role of RNA Polymerase
RNA polymerase is at the heart of the transcription process. This enzyme catalyzes the synthesis of RNA by unwinding the DNA double helix and linking ribonucleotides to form an RNA chain. There are different types of RNA polymerases in eukaryotic cells, specifically RNA polymerase I, II, and III, each responsible for synthesizing different types of RNA.
- RNA polymerase I primarily synthesizes ribosomal RNA (rRNA), which is essential for the formation of ribosomes.
- RNA polymerase II is responsible for synthesizing mRNA and play a central role in gene expression.
- RNA polymerase III synthesizes transfer RNA (tRNA) and small nuclear RNAs, which are crucial for various cellular functions.
The versatility and specificity of RNA polymerases enable cells to respond to various signals and conditions by adjusting the expression of specific genes.
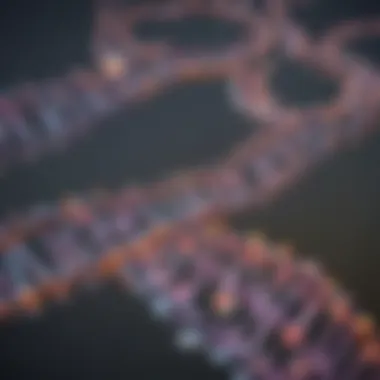
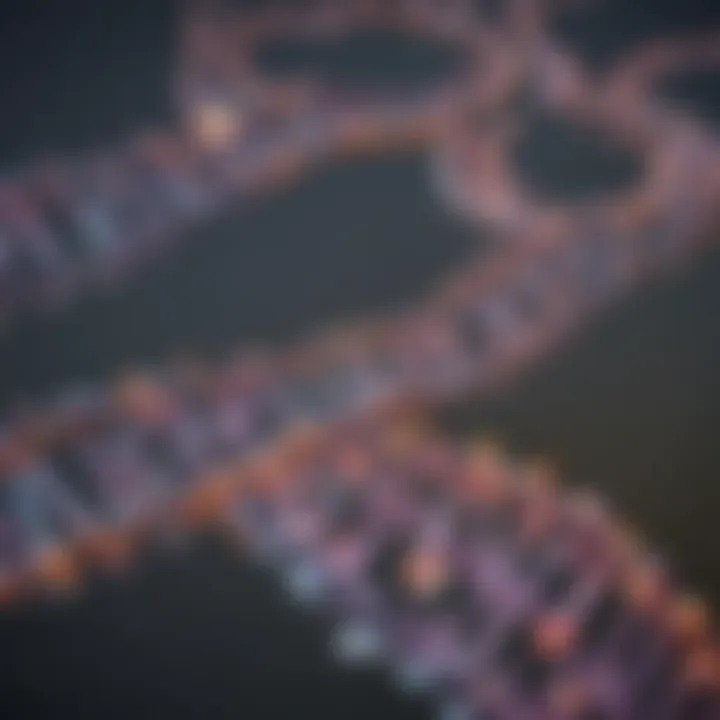
Post-Transcriptional Modifications
After transcription, the newly formed mRNA undergoes several modifications known as post-transcriptional modifications. These alterations are crucial for the stability, transport, and function of mRNA. Common modifications include:
- Capping: A 5’ cap is added, which protects the mRNA from degradation and aids in translation initiation.
- Polyadenylation: A poly-A tail is added to the 3’ end, enhancing the stability of the mRNA and facilitating its export from the nucleus to the cytoplasm.
- Splicing: Introns, or non-coding regions, are removed, while exons, or coding sequences, are joined together to form a continuous coding sequence. This processing allows for different protein isoforms to be generated from a single gene, contributing to the diversity of the proteome.
These modifications significantly influence how efficient the mRNA is at being translated into a protein, and consequently, they play a vital role in cellular regulation.
The transcription process sets the foundation for how genetic information is ultimately expressed within a cell. Understanding these processes allows researchers to explore therapeutic and biotechnological applications more effectively.
Understanding the Genetic Code
Understanding the genetic code is essential in comprehending how mRNA sequences determine the structure of proteins. The genetic code is largely what links the sequence of nucleotides in mRNA to the specific amino acids that eventually compose a protein. Each set of three nucleotides in an mRNA strand—called a codon—corresponds to a particular amino acid or a stop signal during protein synthesis. Thus, understanding this mechanism provides the foundational knowledge necessary for exploring various biotechnological applications and medical innovations, including vaccine development.
The genetic code is nearly universal among all organisms, which signifies its evolutionary importance. By studying the genetic code and its complexities, researchers can develop therapies more effectively and can also better understand genetic diseases and their origins. The implications of grasping the intricacies of the genetic code extend to multiple fields, ranging from genetic engineering to therapeutic applications. It lays a path for future advancements while emphasizing the need for precise interventions in genetic modifications.
Codons and Their Function
Codons are the building blocks of the genetic code, consisting of three nucleotide bases. There are 64 possible codon combinations, which provide instructions for assembling the twenty different amino acids. The significance of individual codons lies in their ability to specify which amino acids to add to a growing polypeptide chain during translation.
When a ribosome encounters a codon on the mRNA, it serves as a signal for tRNA molecules that carry the corresponding amino acid. Each tRNA has an anticodon that pairs with its complementary mRNA codon. This pairing ensures accuracy and contributes to the fidelity of protein synthesis. Moreover, the arrangement of codons along the mRNA dictates the sequence of the resulting protein.
Here are some points to consider regarding codons and their function:
- Codons are read sequentially during translation.
- Each codon corresponds to one amino acid or a stop signal.
- The start codon (AUG) signals the beginning of translation.
Codons provide the essential interface between the nucleic acid sequence and the protein's amino acid sequence, forming the crux of gene expression.
Redundancy and Universality of the Genetic Code
The genetic code exhibits redundancy, meaning multiple codons can encode the same amino acid. For example, leucine is represented by six different codons. This redundancy provides a buffer against potential mutations; a change in one nucleotide may not always alter the resulting amino acid due to this degeneracy. This feature can help in maintaining protein function despite genetic variability.
Furthermore, the universality of the genetic code implies that it is conserved across a vast array of organisms, from bacteria to humans. This aspect facilitates cross-species gene manipulation, enabling scientists to introduce desired traits across different species. The shared code underscores the common ancestry of life on Earth and provides a basis for evolutionary biology studies.
In summary, the redundancy and universality of the genetic code add layers of complexity, importance, and resilience to the biological systems. Recognizing these elements enhances the understanding of genetic research methods and the applications that arise from them.
Translation Process
The translation process is critical in the understanding of how mRNA sequences dictate protein synthesis. It converts the genetic information carried by mRNA into functional proteins, which are essential for numerous cellular functions. This intricate process involves multiple components, each contributing to the overall efficiency and accuracy of protein production.
Ribosomal Structure and Function
Ribosomes play a central role in translation. They serve as the site where protein synthesis occurs. Composed of ribosomal RNA (rRNA) and proteins, ribosomes have two subunits: the large subunit and the small subunit. The small subunit binds to the mRNA, while the large subunit facilitates peptide bond formation between amino acids.
The structure of ribosomes is adapted for their function. The small subunit ensures that the mRNA is read correctly, while the large subunit enables the assembly of amino acids into polypeptide chains. They are also highly conserved across different species, indicating their essential role in biology. Understanding ribosomal structure helps scientists explore how translation can be affected by various factors, such as antibiotics or genetic mutations.
It's important to note that ribosome activity can be influenced by the concentration of amino acids available.
Role of Transfer RNA (tRNA)
Transfer RNA (tRNA) acts as an adapter molecule that brings amino acids to the ribosome during translation. Each tRNA molecule carries a specific amino acid and has an anticodon that pairs with a corresponding codon on the mRNA. This ensures that the correct amino acid is incorporated into the growing polypeptide chain according to the mRNA sequence.
The ability of tRNA to recognize codons enhances the fidelity of protein synthesis. In eukaryotic cells, the process of tRNA maturation involves several steps to ensure functionality. The diversity of tRNA species, which varies between organisms, reflects the complexity of translational processes and how organisms adapt to their environments.
Initiation, Elongation, and Termination of Translation
Translation occurs in three main stages: initiation, elongation, and termination.
- Initiation: This phase begins when the small ribosomal subunit assembles around the start codon on the mRNA. Initiator tRNA then binds to this codon, bringing the first amino acid, typically methionine. The large ribosomal subunit subsequently joins to form a complete ribosome.
- Elongation: During elongation, tRNA molecules sequentially bring amino acids to the ribosome as each codon is read. Peptide bonds are formed between adjacent amino acids, creating a growing polypeptide chain. This process involves several elongation factors that facilitate tRNA binding and movement along the ribosome.
- Termination: When a stop codon is encountered on the mRNA, translation terminates. Release factors bind to the ribosome, prompting it to release the newly synthesized protein and disassemble the ribosomal complex.
Each of these stages is regulated tightly to ensure accurate protein synthesis. Errors during any of these phases can lead to dysfunctional proteins, which may impact cellular health and function. Understanding these processes provides insights into genetic expression and the potential implications for biomedical research.
Amino Acids and Protein Structure
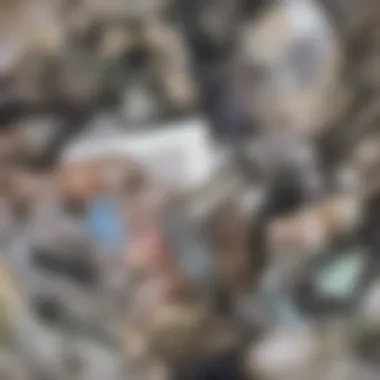
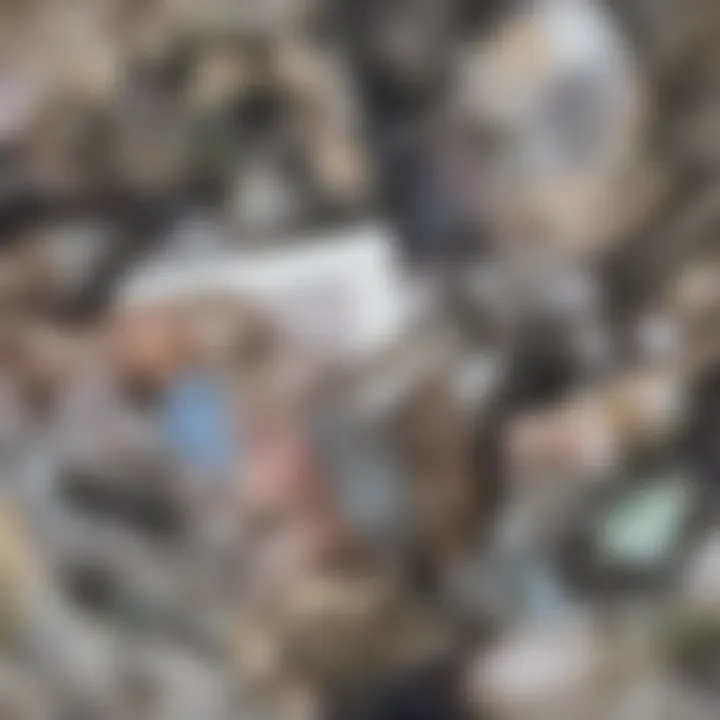
Amino acids are the foundational units of proteins, and understanding their roles is crucial for grasping how proteins function within biological systems. Their diversity and the complex ways they combine contribute significantly to the structure and functionality of proteins. The implications of this diversity are far-reaching, impacting everything from enzyme activity to immune response. This section will delve into the diversity of amino acids, how peptide bonds form between them, and the various levels of protein structure that ultimately determine a protein’s function.
Diversity of Amino Acids
Amino acids are not all the same; there are 20 standard amino acids, each with distinct properties. This diversity is essential because the different side chains or R groups of amino acids can affect the stability and functionality of proteins. Amino acids can be classified based on various criteria, including hydrophilicity, size, and charge. For instance, amino acids like glycine are small and hydrophobic, while others like arginine are larger and positively charged.
The unique combinations of these amino acids lead to vast differences in protein structures and functions. Protein synthesis therefore represents a highly organized method of assembling amino acids to meet specific functional needs. The implications of this diversity are considerable, informing fields like biotechnology and pharmaceuticals. Understanding the specific roles of various amino acids can aid in the design of novel proteins, which could lead to better drug designs and therapeutic solutions.
Peptide Bond Formation
Peptide bonds are the chemical bonds that link amino acids together to form proteins. Each bond forms through a condensation reaction, where a molecule of water is released. This process requires energy and occurs during translation, the next step after transcription in protein synthesis.
The formation of peptide bonds is crucial in maintaining the integrity and sequence of amino acids necessary for proper protein function. A disruption in the bonding could lead to malfunctioning proteins, which may contribute to disease processes. An important aspect to consider is that peptide bonds are relatively stable, which contributes to the overall stability of the protein structure, enabling it to retain its function under various conditions.
Levels of Protein Structure
Primary Structure
The primary structure of a protein is determined by the specific sequence of amino acids. This linear arrangement is crucial; even a single change in the amino acid sequence can drastically alter the protein's properties and functions. For instance, sickle cell anemia is caused by a single substitution in the hemoglobin molecule. Understanding this level of structure is beneficial as it forms the foundation for all further folding and structural arrangements. The primary structure is unique to each protein, which adds to the complexity and diversity in biological systems.
Secondary Structure
The secondary structure refers to local folded structures that form within a protein due to hydrogen bonding between backbone atoms. Common types of secondary structures are alpha helices and beta sheets. The characteristic patterns that emerge at this level are beneficial as they stabilize the protein and dictate how other regions might interact. A specific feature of secondary structure is that it is often repetitive, which allows proteins to maintain stability while allowing flexibility needed for functionality.
Tertiary Structure
The tertiary structure results from the three-dimensional arrangement of multiple secondary structure elements. This means that interactions among side chains lead to compact, defined shapes. Such structures are crucial for the biological activity of enzymes and receptors. The tertiary structure is often characterized by the formation of hydrophobic cores that help to mediate protein stability. Understanding this structure is vital because it can significantly affect how a protein interacts with other molecules, influencing physiological processes.
Quaternary Structure
The quaternary structure refers to the assembly of multiple polypeptide chains, or subunits, into a single functional complex. This type of structure is common in proteins that perform complex functions. One key characteristic is that quaternary structures can often exhibit cooperative behavior; the binding of one molecule can affect the binding properties of another. For example, hemoglobin’s ability to carry oxygen is due to its quaternary structure, with four heme groups working together effectively. However, the quaternary structure introduces additional complexity and can be sensitive to changes in environmental conditions, which may affect protein function.
Understanding the structure of proteins is not merely academic; it holds implications for drug design, synthetic biology, and understanding diseases.
The study of amino acids and protein structure, therefore, feeds into a larger context that has profound implications for science and medicine.
Applications of mRNA Technology
The advent of mRNA technology represents a significant shift in biotechnology, shaping various domains, including medicine, agriculture, and genetic engineering. This section examines the pivotal role of mRNA applications, highlighting their benefits and considerations in diverse fields. The importance of this technology cannot be overstated, as it opens new avenues for innovative treatments and solutions.
Role in Vaccine Development
mRNA vaccines have taken center stage in the fight against infectious diseases. These vaccines utilize synthetic mRNA to instruct cells to produce proteins resembling those from pathogens, prompting an immune response without causing disease. The success of the Pfizer-BioNTech and Moderna COVID-19 vaccines exemplifies how rapidly mRNA technology can be integrated into health protocols.
Some key benefits include:
- Rapid Development: mRNA vaccines can be designed quickly in response to emerging diseases.
- Safety Profile: There is no live pathogen in mRNA vaccines, reducing the risk of disease transmission.
- Efficacy: Clinical trials have shown strong immune responses following administration.
However, challenges exist, such as the need for cold storage and public acceptance. As more data become available, addressing these concerns will be crucial for broad vaccination strategies.
Therapeutic Applications
Beyond vaccines, mRNA technology is being explored for therapeutic applications in treating diseases, including cancer and genetic disorders. The approach involves harnessing mRNA to trigger the body’s immune response or correct genetic mutations.
Key considerations in therapeutic applications include:
- Personalized Medicine: mRNA therapies can be tailored to individual genetic makeups, offering targeted treatments.
- Broader Scope: They can potentially treat a wide range of conditions, from rare genetic disorders to complex diseases like cancer.
- Continuous Research: Ongoing studies aim to optimize delivery methods and improve stability.
As this field grows, the potential for mRNA technology to revolutionize treatment across various medical fields increases significantly.
mRNA in Genetic Engineering
mRNA technology also plays a pivotal role in genetic engineering. It allows for precise manipulation of gene expression, enabling researchers to address specific genes associated with diseases.

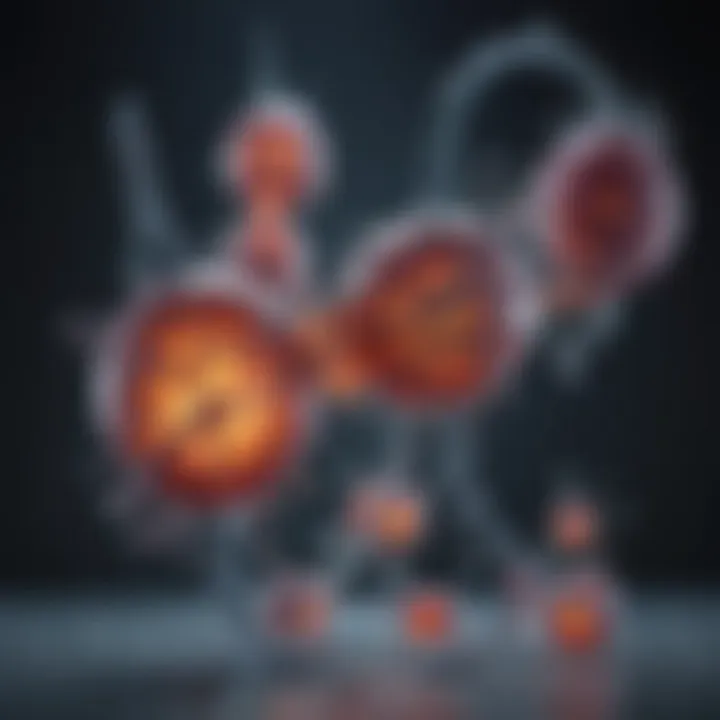
The implications of mRNA in genetic engineering include:
- Efficient Gene Editing: Tools like CRISPR can use mRNA to guide genome editing more effectively.
- Synthetic Biology: Researchers can program cells with synthetic mRNA to produce desired proteins, enhancing industrial and research processes.
- Ethical Implications: As this technology advances, it raises crucial ethical questions regarding genetic modifications and potential impacts on biodiversity.
In summary, the applications of mRNA technology extend far beyond vaccines. The potential in therapy and genetic engineering shows promise for advancing health and agricultural solutions. As research continues, it is imperative to navigate the ethical landscape while harnessing the benefits of this groundbreaking technology.
"mRNA technology not just addresses immediate health challenges but also paves the way for future innovation in biotechnology and medicine."
The possibilities are vast, and the impact of mRNA technology will likely shape our understanding of health and disease in unprecedented ways.
Challenges and Ethical Considerations in mRNA Research
As the field of mRNA research expands, it offers immense potential for innovation in medicine and biotechnology. However, with this potential come significant challenges and ethical considerations that must be addressed. Understanding these issues is crucial not only for researchers but also for policymakers and the public.
Regulatory Concerns
The rapid development of mRNA technology has outpaced existing regulatory frameworks. This raises several important questions regarding safety, efficacy, and long-term impacts of mRNA products. Regulatory bodies, such as the Food and Drug Administration (FDA) in the United States, are tasked with ensuring that new therapies meet rigorous standards. However, the unprecedented speed of mRNA vaccine deployment during the COVID-19 pandemic has prompted discussions on whether current regulations are sufficient.
Some key regulatory challenges include:
- Quality Control: Ensuring that mRNA is consistently produced with high purity and integrity is vital. Any variation can lead to differences in vaccine efficacy.
- Clinical Trials: The approach to clinical trials for mRNA-based therapies needs refinement. Rapid trials may overlook long-term effects that can arise years after administration.
- Post-Market Surveillance: Ongoing monitoring of mRNA products after they are approved is essential. This includes collecting data on rare side effects that might not be evident during initial trials.
Dealing with these regulatory challenges is a complex process. Strong collaboration between scientists, ethicists, and regulatory agencies is essential to formulate comprehensive strategies that protect public health while fostering innovation.
Public Perception of mRNA Applications
Public understanding and perception of mRNA applications also play a critical role in the advancement of this technology. There have been concerns and misinformation regarding the safety and effectiveness of mRNA vaccines. These perceptions can significantly influence vaccine uptake and public health initiatives.
Factors affecting public perception include:
- Misinformation: The internet has made it easier for misinformation to spread. Erroneous claims about the effects of mRNA technology can lead to hesitation or refusal to receive vaccines.
- Transparency: Clear communication from health organizations about how mRNA works and its benefits can help build trust. Acknowledging concerns and addressing them honestly is crucial.
- Science Literacy: Increasing the public's understanding of genetic science can help demystify mRNA technology. Educational initiatives may play an important role in fostering a scientifically informed populace.
Ultimately, the success of mRNA applications hinges not just on scientific breakthroughs but also on the public's acceptance and understanding of these innovations. Addressing these challenges requires a concerted effort from scientists, regulators, and educators to ensure that this powerful tool is used responsibly and effectively.
"The potential impact of mRNA technology is immense, but we must navigate the complexities of regulation and public perception carefully to realize its benefits fully."
Future Directions in mRNA Research
mRNA research is a rapidly evolving field with significant implications for both science and medicine. As we expand our understanding of mRNA's role in protein synthesis, it is critical to explore future directions that can enhance its applications. This exploration could lead to groundbreaking advancements, shaping how we approach vaccine development, disease treatment, and genetic therapies.
Advancements in Delivery Mechanisms
One of the most pressing challenges in mRNA technology is developing effective delivery mechanisms. Current methods, such as lipid nanoparticles, have proven useful but still show limitations in specificity and efficiency. Future advancements may include courier systems that enhance cellular uptake and ensure targeted delivery.
- Nanoparticle Engineering: Modified nanoparticles may improve stability and reduce immune responses, allowing for higher doses of mRNA.
- Viral Vectors: Utilizing engineered viruses as delivery systems can optimize integration into host cells, leading to sustained expression of the desired proteins.
- Molecular Conjugates: Linking mRNA to specific targeting molecules can change how mRNA reaches specific tissues or cells, enhancing delivery efficiency.
These innovations could revolutionize treatments for various diseases, including cancer and genetic disorders, ensuring mRNA achieves its therapeutic potential. The end goal is to create precise delivery systems that can confidently navigate biological barriers.
Potential Unexplored Areas
While mRNA applications are growing, there remain unexplored areas that offer promising avenues for research. Identifying and investigating these areas can lead to transformative discoveries, greatly beneficial in medicine and biotechnology.
- Single-cell Transcriptomics: Understanding mRNA expression at the single-cell level can unlock insights into cellular diversity and responses to therapies.
- Synthetic Biology: Designing synthetic mRNA with tailored characteristics can enhance its efficacy in various applications, from regenerative medicine to personalized treatments.
- Environmental Impact: Investigating how mRNA technology interacts with the environment may provide insights into its adaptability and long-term effects on ecosystems.
The potential of mRNA research extends beyond human health, offering insights that may redefine our approach to biology itself.
Epilogue
In this article, we investigate the pivotal role of mRNA in determining the amino acid sequence, which is essential for protein synthesis. The conclusion encapsulates the critical insights derived from our exploration of mRNA, emphasizing various aspects related to its functionality and implications in biotechnology and medicine.
Summary of Key Points
- mRNA serves as a carrier of genetic information from DNA to the ribosomes, where proteins are synthesized.
- The genetic code comprises codons that dictate the specific sequence of amino acids, essential for building proteins.
- The redundancy and universality of the genetic code contribute to the diversity of life and evolutionary processes.
- mRNA technology has gained significant attention for its applications in vaccine development and therapeutic strategies, showcasing its potential to address various health challenges.
- Challenges surrounding regulatory concerns and public perception influence the pace of mRNA research and its implementation in healthcare.
The Importance of Continued Research
Ongoing advancements in mRNA research are crucial for several reasons. Firstly, understanding the nuances of mRNA dynamics can lead to more effective vaccines and therapies. For instance, the success of mRNA vaccines during the global pandemic underscored the need for deeper knowledge in this area. Researchers must continue to explore innovative delivery mechanisms to enhance efficacy and safety.
Furthermore, dissecting potential unexplored areas, such as the role of mRNA in non-coding sequences and applications in gene therapy, could open new avenues for medical breakthroughs. As we increase our grasp of mRNA's capabilities and limitations, we pave the way for transforming therapeutics and biotechnology.
"Research is the key to unlock future potential; continued inquiry will shape our healthcare landscape and broaden our understanding of biological systems."
In essence, the examination of mRNA and its influence on amino acid sequencing is not merely an academic exercise. It carries with it the prospect of profound implications for human health and technological advancement. Continued investment in research and ethical considerations will assure that these benefits are realized with caution and responsibility.